Ying Zhang, Qiang Liu, Chenyang Mei, Desheng Zeng, Qingzhong Huang, Xinliang Zhang, "Proposal and demonstration of a controllable Q factor in directly coupled microring resonators for optical buffering applications," Photonics Res. 9, 2006 (2021)

Search by keywords or author
- Photonics Research
- Vol. 9, Issue 10, 2006 (2021)
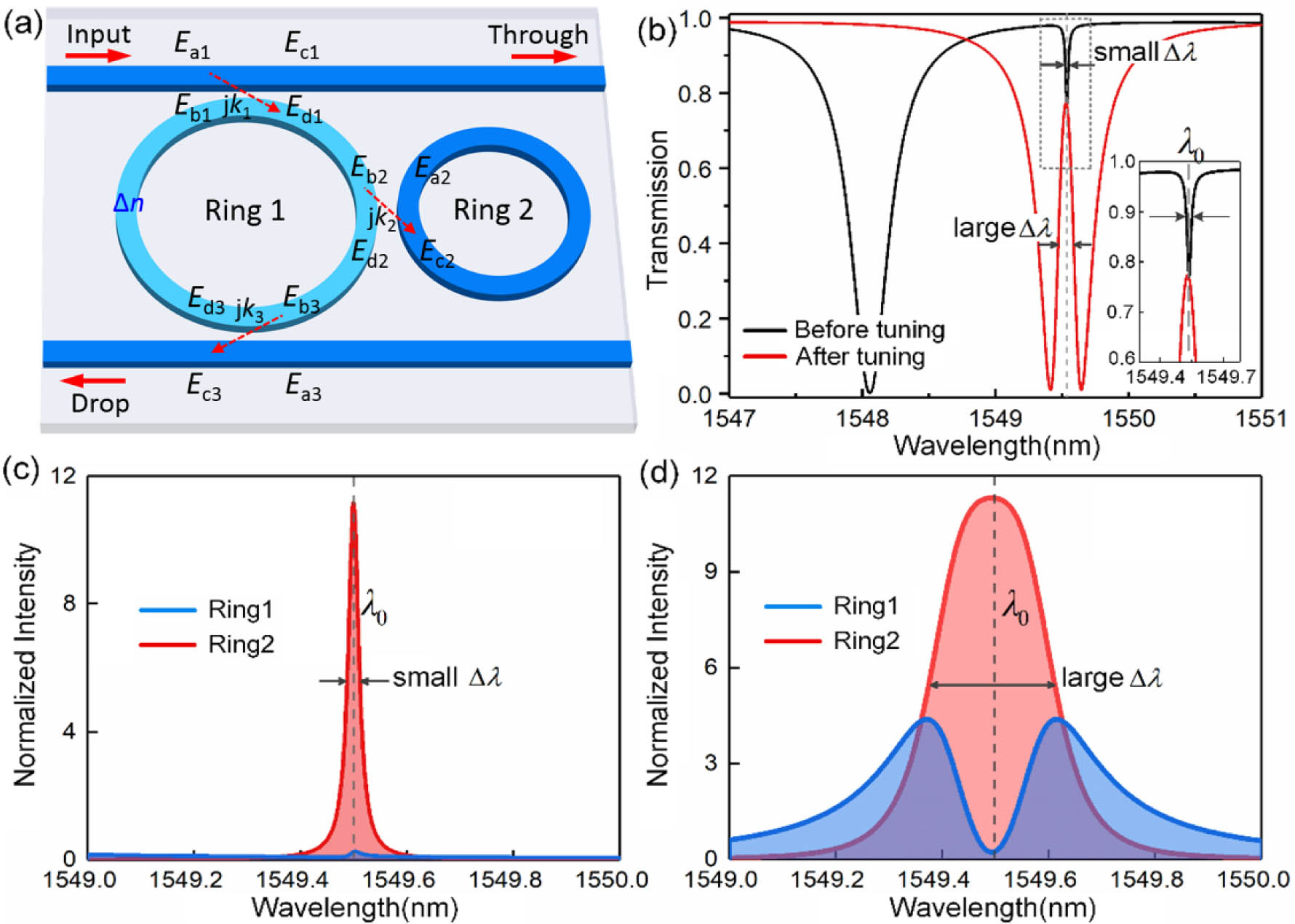
Fig. 1. (a) Schematic configuration of the Q -factor controllable structure. (b) Transmission spectra at the through port with/without refractive index change, illustrating the variation of Δ λ ; inset: zoom-in of the spectra around λ 0 . Optical intensities in Ring 1 and Ring 2 (c) before tuning and (d) after tuning.
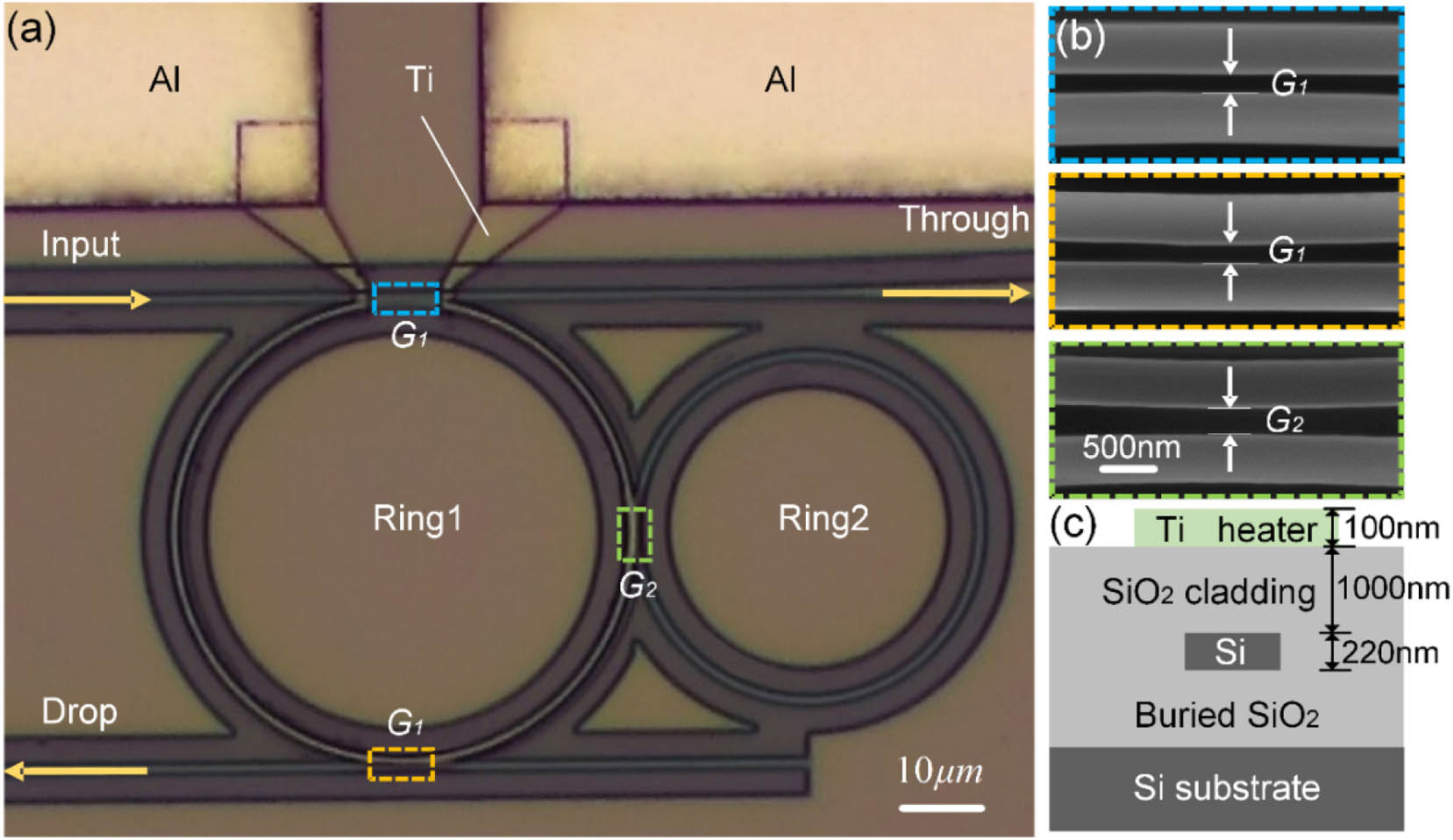
Fig. 2. (a) Microscope image of the fabricated Q -factor controllable system. (b) Scanning electron microscopy images of different coupling regions before the SiO 2 layer was deposited. (c) Schematic of waveguide cross section in Ring 1 with a Ti heater on the top.
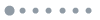
Fig. 3. (a) Experimental transmission spectra (circle dots) and theoretical fits (solid curves) of the fabricated device. Inset: zoom-in of the high-Q resonance. (b) Normalized intensity in Ring 2 theoretically simulated using the fitting parameters.
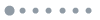
Fig. 4. (a) Experimental transmission spectra (circle dots) and theoretical fits (solid curves) of the fabricated device with G 2 of 160 nm. (b) Normalized intensity in Ring 2 theoretically simulated using the fitting parameters.
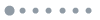
Fig. 5. Experimental transmission spectra (circle dots) and theoretical fits (solid curves) of the devices with different G 2 in the (a) low-Q state and (b) high-Q state.
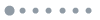
Fig. 6. Normalized intensity spectra in Ring 2 for different k 2 ranging from 0.12 to 0.39.
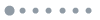
Fig. 7. (a) Time delay of the system as a function of wavelength detuning in the high-Q state and low-Q state, theoretically simulated using the extracted parameter values of the device shown in Fig. 3 . The maximum time delay and time advance are provided at points P1 and P2, respectively. (b) The measured optical pulses output from the system in the low-Q state (P1) and reference waveguide (R). The time delay is obtained by comparing the two pulses in the time domain. (c) The measured phase shift response (red circles) and its fitting curve (red line) of the system in the high-Q state. The time delay (black line) is deduced from the fitted phase shift using τ d = − d φ / d ω . (d) The time delay and FWHM (Δ λ ) of the system in the low-Q state and high-Q state for different G 2 . The system produces slow light and fast light (time delay having negative values) in the low-Q state and high-Q state, respectively.
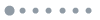
Fig. 8. Simulated dynamics of the intra-cavity and output optical intensities of the system under control. (a) Normalized intensity in Ring 1/Ring 2 with S control (storing at t = 65 ps , dashed lines), or S + R control (storing at t = 65 ps and releasing at t = 100 ps , solid lines). When Ring 1 is dynamically tuned by S control, the system is immediately switched to the high-Q state and stores light in Ring 2. Then, as R control is applied, the system returns to the low-Q state and releases light from Ring 2. (b) Optical intensities at the output ports (through: solid lines; drop: dashed lines) with S + R control (storing at t = 65 ps , different releasing time). When S control is applied, the pulse intensities at the output ports become low, since the light is trapped in Ring 2. (c) Optical intensities at the output ports (through: solid lines; drop: dashed lines) of different systems, whose curves are offset for clarity. S control is applied for storing, just when the optical intensity in Ring 2 reaches the maximum. (d) The peak intensity of output pulse at the drop port as a function of storage time. The system denoted by k 2 = 0.10 is identical to that in Fig. 3 , while the systems denoted by k 2 = 0.12 , 0.17, 0.24, 0.31, 0.39 are identical to those in regions (i)–(v) in Fig. 5 , respectively, except for the slow tuning. Here, these systems are assumed to be dynamically tunable.
|
Table 1. Comparison of Various Q -Factor Controllable Systems
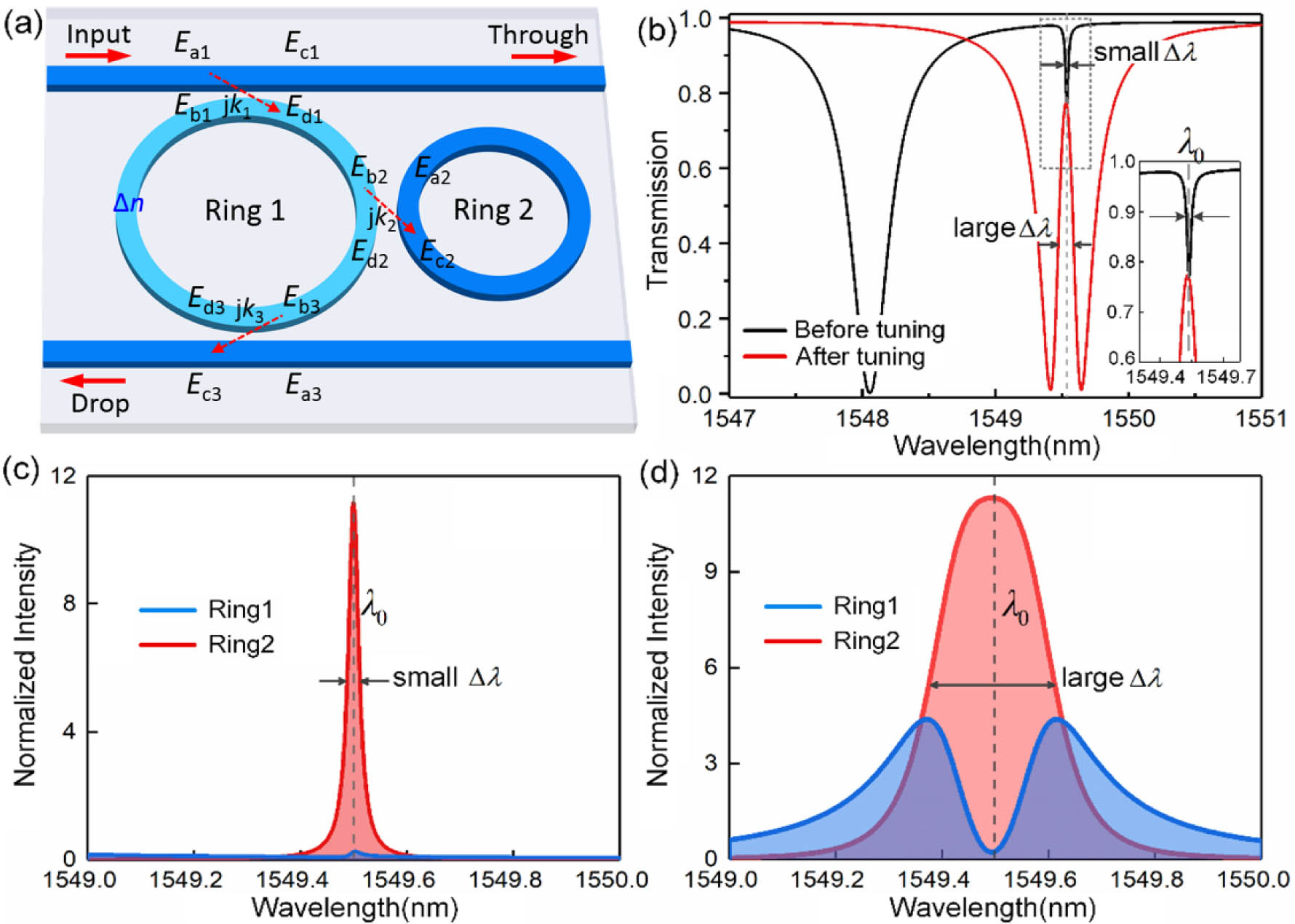
Set citation alerts for the article
Please enter your email address