
- Photonics Research
- Vol. 9, Issue 10, 2006 (2021)
Abstract
1. INTRODUCTION
Optical resonators [1] have been widely used in various devices, such as light sources [2,3], filters [4,5], modulators [6,7], switches [8,9], and buffers [10–12], which are essential components for optical communications and optical signal processing. As a characteristic parameter, the
To date, there are fundamentally three approaches to control the
In this paper, we present and investigate systematically a
Sign up for Photonics Research TOC. Get the latest issue of Photonics Research delivered right to you!Sign up now
2. DEVICE STRUCTURE AND THEORY
Figure 1(a) shows a schematic configuration of the presented
Figure 1.(a) Schematic configuration of the
Consequently, the transfer functions at the through port (
To analyze this system, we have calculated the transmission spectra at the output port using the previous equations. There are some basic considerations to choose parameter values. First, Ring 1 is designed as a low-
Figure 1(b) shows the transmission spectra at the through port before and after the tuning of
To provide more insight, we have further calculated the optical intensities in Ring 1 and Ring 2 before and after tuning. Figures 1(c) and 1(d) illustrate the variation of internal optical intensity in Ring 2 after tuning, illustrating the controllable
3. EXPERIMENTAL RESULTS
A. Device Fabrication
A proposed structure was fabricated on a silicon-on-insulator platform for example, as shown in Fig. 2. The top silicon layer and buried oxide layer are 220 nm and 2 μm in thickness, respectively. The waveguides and rings were all defined using e-beam lithography, followed by inductively coupled plasma etching. Simultaneously, grating couplers were constructed to couple the device with input/output fiber for TE polarization. The straight and bent waveguides all have a height of 220 nm and a width of 450 nm. The radii of Ring 1 and Ring 2 are about 28 μm and 21 μm, respectively. The spacing gap between Ring 1 and waveguide (Ring 2) is
Figure 2.(a) Microscope image of the fabricated
B. Control of
Figure 3(a) illustrates the normalized transmission spectra of the fabricated device at the through port for different heating powers. In the beginning, without heating, the transmission spectrum exhibits two separated valleys. The left resonant valley from Ring 1 is broadband and has a high extinction ratio. In contrast, the right valley from Ring 2 has an FWHM of only 27 pm, corresponding to a
Figure 3.(a) Experimental transmission spectra (circle dots) and theoretical fits (solid curves) of the fabricated device. Inset: zoom-in of the high-
To further study the
Figure 4.(a) Experimental transmission spectra (circle dots) and theoretical fits (solid curves) of the fabricated device with
Figures 5(a) and 5(b) show the measured transmission spectra of the system with a changed
Figure 5.Experimental transmission spectra (circle dots) and theoretical fits (solid curves) of the devices with different
The coupling coefficient
Figure 6.Normalized intensity spectra in Ring 2 for different
Table 1 summarizes and compares the existing
Comparison of Various
Mechanism | Structure | Material | Control Method | Reference | |
---|---|---|---|---|---|
Control of loss/coupling | PhC cavity | AO tuning, off chip | 52,000–210,000 | [ | |
MRR integrated with an SOA | InP-InGaAsP | Electrical pumping, on chip | 13,000–32,000 | [ | |
MRR coupled with a waveguide | TO tuning, on chip | 9000–96,000 | [ | ||
Interference between a resonator and a feedback waveguide | MRR with interferometric couplers | TO tuning, on chip | 2200–15,500 | [ | |
PhC cavity–waveguide–mirror system | AO tuning, off chip | 3000–12,000 | [ | ||
PhC cavity–waveguide–mirror system | AO tuning, off chip | 3800–22,000 | [ | ||
PhC cavity–waveguide–mirror system | GaAs-AlGaAs | AO tuning, off chip | 7900–40,000 | [ | |
Interference between coupled resonators | Two indirectly coupled MRRs | EO tuning, on chip | 6000–20,000 | [ | |
Two indirectly coupled MRRs | AO tuning, off chip | 23,000–53,000 | [ | ||
Laterally coupled vertical cavities | GaAs-AlGaAs | TO tuning, off chip | 1650–1900 | [ | |
Two directly coupled MRRs | TO tuning, on chip | 16,400–56,5002800–21,800 | Our work |
The
The
4. APPLICATIONS IN OPTICAL BUFFERING
A. Tunable Slow and Fast Light
Below, we explore the applications of the proposed system in optical buffering. It is known that the group delay can be calculated by
Figure 7.(a) Time delay of the system as a function of wavelength detuning in the high-
Consequently, we measure the time delay of the system in the low-
B. Optical Storage
The
Then, based on the theoretical model, we have analyzed the light storing and releasing in the systems which are identical to the realized devices, assuming Ring 1 is dynamically tunable. Figure 8(a) shows the dynamics of normalized intensity in Ring 1/Ring 2 with storing (S) control followed by releasing (R) control or not. The optical intensities are normalized to the peak intensity of the input pulse. The system is initially in the low-
Figure 8.Simulated dynamics of the intra-cavity and output optical intensities of the system under control. (a) Normalized intensity in Ring 1/Ring 2 with S control (storing at
The time delay/advance of the fabricated devices is of the order of tens of picoseconds, due to the limited
5. CONCLUSIONS
In summary, we have demonstrated a
References
[1] K. J. Vahala. Optical microcavities. Nature, 424, 839-846(2003).
[2] J. T. Clarke, J. C. Gerard, D. Grodent, S. Wannawichian, J. Gustin, J. Connerney, F. Crary, M. Dougherty, W. Kurth, S. W. Cowley, E. J. Bunce, T. Hill, J. Kim. A continuous-wave Raman silicon laser. Nature, 433, 725-728(2005).
[3] L. Liu, T. Spuesens, G. Roelkens, D. V. Thourhout, P. Regreny, P. R. Romeo. A thermally tunable III–V compound semiconductor microdisk laser integrated on silicon-on-insulator circuits. IEEE Photonics Technol. Lett., 22, 1270-1272(2010).
[4] Y. Ren, D. Perron, F. Aurangozeb, Z. Jiang, M. Hossain, V. Van. Silicon photonic vernier cascaded microring filter for broadband tunability. IEEE Photonics Technol. Lett., 31, 1503-1506(2019).
[5] Q. Huang, J. Yu. Coherent interaction between two orthogonal travelling-wave modes in a microdonut resonator for filtering and buffering applications. Opt. Express, 22, 25171-25182(2014).
[6] Q. Xu, B. Schmidt, S. Pradhan, M. Lipson. Micrometre-scale silicon electro-optic modulator. Nature, 435, 325-327(2005).
[7] X. Xiao, H. Xu, X. Li, Y. Hu, K. Xiong. 25 Gbit/s silicon microring modulator based on misalignment-tolerant interleaved PN junctions. Opt. Express, 20, 2507-2515(2012).
[8] Y. Vlasov, W. M. J. Green, F. Xia. High-throughput silicon nanophotonic wavelength-insensitive switch for on-chip optical networks. Nat. Photonics, 2, 242-246(2008).
[9] Q. Huang, X. Zhang, J. Xia, J. Yu. Systematic investigation of silicon digital 1 × 2 electro-optic switch based on a microdisk resonator through carrier injection. Appl. Phys. B, 105, 353-361(2011).
[10] F. Xia, L. Sekaric, Y. Vlasov. Ultracompact optical buffers on a silicon chip. Nat. Photonics, 1, 65-71(2006).
[11] Q. Huang, G. Song, J. Chen, Z. Shu, J. Yu. Proposal and fabrication of an electrooptically controlled multimode microresonator for continuous fast-to-slow light tuning. IEEE Photonics J., 6, 2201011(2014).
[12] A. W. Elshaari, A. Aboketaf, S. F. Preble. Controlled storage of light in silicon cavities. Opt. Express, 18, 3014-3022(2010).
[13] K. Shtyrkova, P. T. Callahan, N. Li, E. S. Magden, A. Ruocco, D. Vermeulen, F. X. Kartner, M. R. Watts, E. P. Ippen. Integrated CMOS-compatible
[14] L. Chen, N. Sherwood-Droz, M. Lipson. Compact bandwidth-tunable microring resonators. Opt. Lett., 32, 3361-3363(2007).
[15] H. Shoman, H. Jayatilleka, A. H. K. Park, A. Mistry, N. A. F. Jaeger, S. Shekhar, L. Chrostowski. Compact wavelength- and bandwidth-tunable microring modulator. Opt. Express, 27, 26661-26675(2019).
[16] Y. Liu, A. Wichman, B. Isaac, J. Kalkavage, J. Klamkin. Ultra-low-loss silicon nitride optical beamforming network for wideband wireless applications. IEEE J. Sel. Top. Quantum Electron., 24, 8300410(2018).
[17] Q. Xu, P. Dong, M. Lipson. Breaking the delay-bandwidth limit in a photonic structure. Nat. Phys., 3, 406-410(2007).
[18] T. Tanabe, M. Notomi, H. Taniyama, E. Kuramochi. Dynamic release of trapped light from an ultrahigh-
[19] V. M. Menon, W. Tong, S. R. Forrest. Control of quality factor and critical coupling in microring resonators through integration of a semiconductor optical amplifier. IEEE Photonics Technol. Lett., 16, 1343-1345(2004).
[20] M. J. Strain, C. Lacava, L. Meriggi, I. Cristiani, M. Sorel. Tunable Q-factor silicon microring resonators for ultra-low power parametric processes. Opt. Lett., 40, 1274-1277(2015).
[21] Y. Tanaka, J. Upham, T. Nagashima, T. Sugiya, T. Asano, S. Noda. Dynamic control of the
[22] J. Upham, Y. Tanaka, T. Asano, S. Noda. Dynamic increase and decrease of photonic crystal nanocavity
[23] J. Upham, H. Inoue, Y. Tanakmodesa, W. Stumpf, K. Kojima, T. Asano, S. Noda. Pulse capture without carrier absorption in dynamic
[24] S. Manipatruni, C. B. Poitras, Q. Xu, M. Lipson. High-speed electro-optic control of the optical quality factor of a silicon microcavity. Opt. Lett., 33, 1644-1646(2008).
[25] Y. Zhang, Q. Huang, Q. Liu. Controllable optical quality factor in a two-ring-two-bus structure. Proc. SPIE, 11761, 1176104(2021).
[26] S. J. Emelett, R. A. Soref. Synthesis of dual-microring-resonator crossconnect filters. Opt. Express, 13, 4439-4456(2005).
[27] S. J. Emelett, R. A. Soref. Analysis of dual-microring-resonator cross-connect switches and modulators. Opt. Express, 13, 7840-7853(2005).
[28] Q. Xu, J. Shakya, M. Lipson. Direct measurement of tunable optical delays on chip analogue to electromagnetically induced transparency. Opt. Express, 14, 6463-6468(2006).
[29] J. Liu, H. Tian, E. Lucas, A. S. Raja, G. Lihachev, R. N. Wang, J. He, T. Liu, M. H. Anderson, W. Weng, S. A. Bhave, T. J. Kippenberg. Monolithic piezoelectric control of soliton microcombs. Nature, 583, 385-390(2020).
[30] C. Wang, M. Zhang, X. Chen, M. Bertrand, A. Shams-Ansari, S. Chandrasekhar, P. Winzer, M. Loncar. Integrated lithium niobate electro-optic modulators operating at CMOS-compatible voltages. Nature, 562, 101-104(2018).
[31] L. Chang, W. Xie, H. Shu, Q. F. Yang, B. Shen, A. Boes, J. D. Peters, W. Jin, C. Xiang, S. Liu, G. Moille, S. P. Yu, X. Wang, K. Srinivasan, S. B. Papp, K. Vahala, J. E. Bowers. Ultra-efficient frequency comb generation in AlGaAs-on-insulator microresonators. Nat. Commun., 11, 1331(2020).
[32] Y. Zheng, J. Yang, Z. Shen, J. Cao, X. Chen, X. Liang, W. Wan. Optically induced transparency in a micro-cavity. Light Sci. Appl., 5, e16072(2016).
[33] C. Wang, X. Jiang, G. Zhao, M. Zhang, C. W. Hsu, B. Peng, A. D. Stone, L. Yang. Electromagnetically induced transparency at a chiral exceptional point. Nat. Phys., 16, 334-340(2020).
[34] S. Chen, H. Francis, C.-H. Ho, Y.-R. Wang, K.-J. Che, M. Hopkinson, C.-Y. Jin. Control of quality factor in laterally coupled vertical cavities. IET Optoelectron., 14, 100-103(2020).
[35] J. Xie, L. Zhou, Z. Li, J. Wang, J. Chen. Seven-bit reconfigurable optical true time delay line based on silicon integration. Opt. Express, 22, 22707-22715(2014).
[36] W. Jiang, L. Xu, Y. Liu, Y. Chen, X. Liu, Y. Yu, Y. Yu, X. Zhang. Optical all-pass filter in silicon-on-insulator. ACS Photonics, 7, 2539-2546(2020).
[37] M. F. Yanik, W. Suh, Z. Wang, S. Fan. Stopping light in a waveguide with an all-optical analog of electromagnetically induced transparency. Phys. Rev. Lett., 93, 233903(2004).
[38] M. Li, L. Wang, X. Li, X. Xiao, S. Yu. Silicon intensity Mach–Zehnder modulator for single lane 100 Gb/s applications. Photon. Res., 6, 109-116(2018).
[39] C.-H. Dong, Z. Shen, C.-L. Zou, Y.-L. Zhang, W. Fu, G.-C. Guo. Brillouin-scattering-induced transparency and nonreciprocal light storage. Nat. Commun., 6, 6193(2015).
[40] T. Qin, J. Yang, F. Zhang, Y. Chen, D. Shen, W. Liu, L. Chen, X. Jiang, X. Chen, W. Wan. Fast- and slow-light-enhanced light drag in a moving microcavity. Commun. Phys., 3, 118(2020).
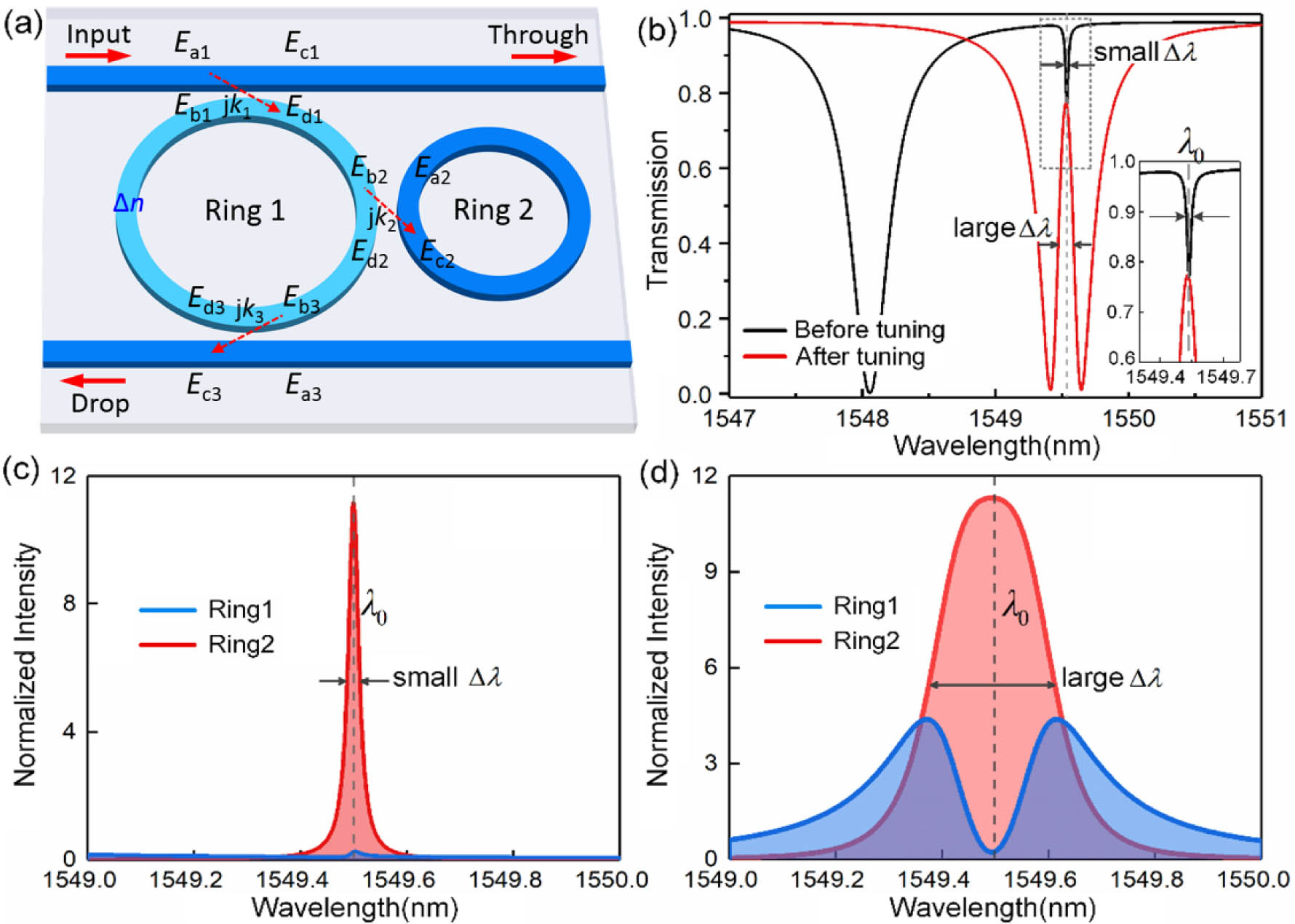
Set citation alerts for the article
Please enter your email address