Author Affiliations
1NanoTech Lab, Department of Electrical Engineering, Information Technology University (ITU) of the Punjab, Lahore 54600, Pakistan2Department of Mechanical Engineering, Pohang University of Science and Technology (POSTECH), Pohang 37673, Republic of Korea3Department of Chemical Engineering, Pohang University of Science and Technology (POSTECH), Pohang 37673, Republic of Korea4POSCO-POSTECH-RIST Convergence Research Center for Flat Optics and Metaphotonics, Pohang 37673, Republic of Korea5National Institute of Nanomaterials Technology (NINT), Pohang 37673, Republic of Korea6e-mail: muhammad.zubair@itu.edu.pk7e-mail: kashif.riaz@itu.edu.pk8e-mail: qasim.mehmood@itu.edu.pkshow less
Fig. 1. Working principle of the single-layered all-dielectric diatomic metasurface and optical setup. (a) Schematic image of the working principle of the proposed diatomic metasurface introducing a giant chiro-optical effect in the visible regime. (b) SEM image of a portion of the fabricated diatomic metasurface. The scale bar is (i) 2 μm; (ii) 0.75 μm; (iii) 0.25 μm. (c) The characterization setup of the designed dielectric metasurface for giant chiro-optical effect. An arc lamp housing is used as the light source. A linear polarizer is used to linearly polarize the input beam, passing through a QWP to produce LHCP or RHCP light. The OL focuses on the polarized light, which illuminates the sample. The transmitted light beam from the sample further passes through another OL, QWP, and polarizer. Finally, the results are recorded by using a spectrometer.
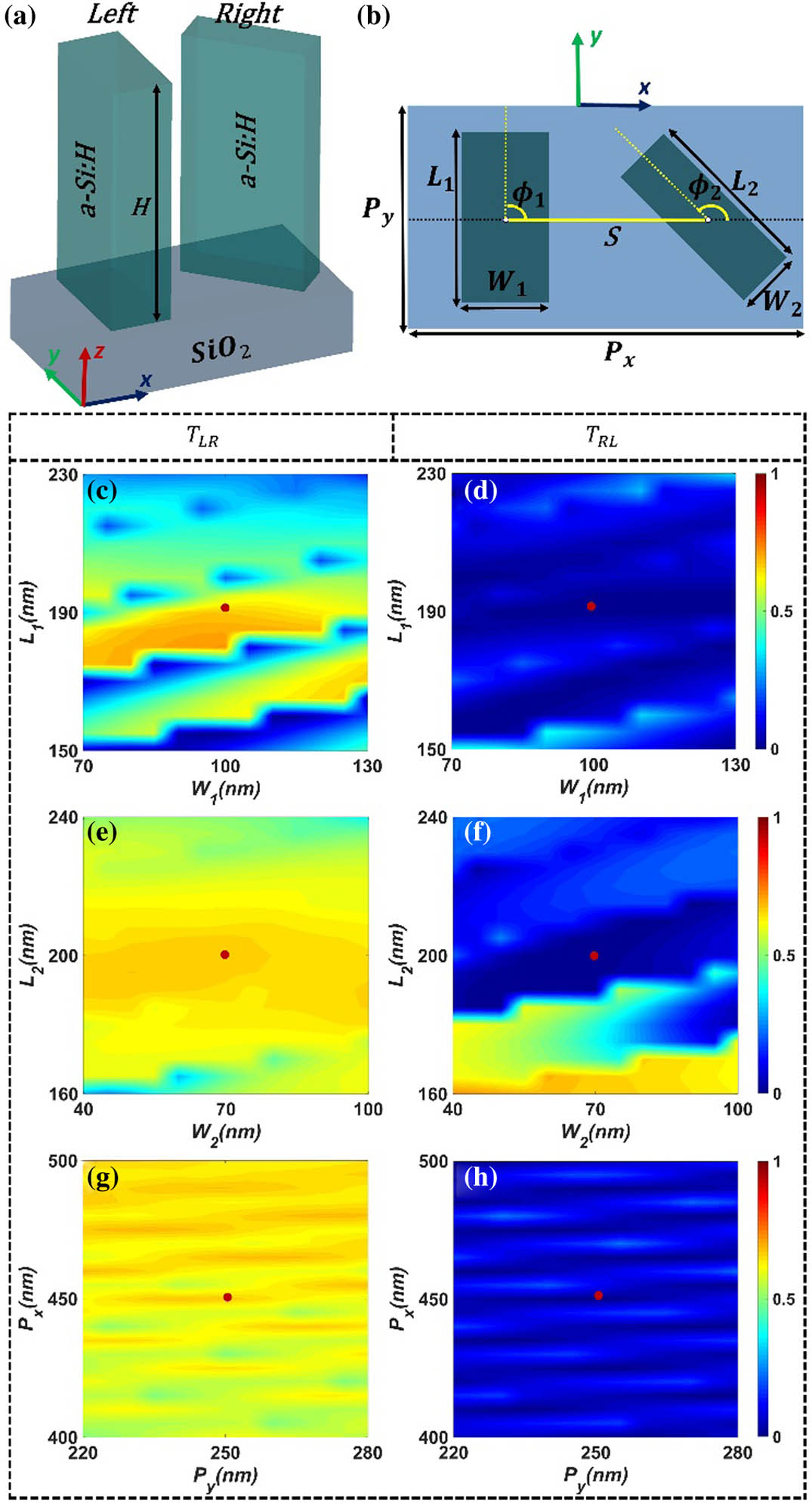
Fig. 2. Optimization of the building block of the diatomic metasurface. (a) 3D perspective view of the building block of the metasurface containing a pair of distinct a-Si:H-based nanofins with a relative angle (Δϕ) of 45 deg to break mirror symmetry with respect to the metasurface plane; (b) top view of the optimized building block of the metasurface with structural parameters of width1 W1=100 nm, length1 L1=195 nm, width2 W2=70 nm, length2 L2=200 nm, displacement S=227.5 nm, periodicity in the x direction Px=455 nm, periodicity in the y direction Py=255 nm, and height H=400 nm. The strong chiro-optical effect depends on the following parameters: the periodicity of the building block, the local displacement between nanofins, which depends on the length and width of the nanofin, and the relative rotation angle between the nanofins along the plane of the metasurface. The simulated cross-polarized transmittance parameter with varying length and width of left nanofin while keeping all other parameters (S,Px,Py, and H) fixed for (c) RHCP and (d) LHCP illumination at the wavelength of 633 nm. The red circle shows the chosen dimensions (L1×W1) are 195 and 100 nm. The simulated cross-polarized transmittance parameter with varying length and width of the right nanofin while keeping all other parameters fixed for (e) RHCP and (f) LHCP illumination at the wavelength of 633 nm. The chosen dimensions (L2×W2) are 200 and 70 nm. The cross-polarized transmittance parameter with a varying periodicity of the building block in the x and y directions while keeping all other parameters fixed for (g) RHCP and (h) LHCP illumination at the wavelength of 633 nm.
Fig. 3. Representation of parameters of the Jones matrix for designed metasurface in transmission and reflection. The simulated coefficients of the Jones matrix in transmission (TLR, LHCP transmission/RHCP incidence; TRL, RHCP transmission/LHCP incidence; TRR, RHCP transmission/RHCP incidence; TLL, LHCP transmission/LHCP incidence) of the metasurface for illumination in the (a) forward and (b) backward directions. Similarly, the measured transmission coefficients are illustrated for illumination in the (d) forward and (e) backward directions. The (c) simulated and (f) measured AT parameters comparing forward and backward propagation. The simulated reflectance coefficients of the Jones matrix are illustrated for illumination in the (g) forward and (h) backward directions. The CD for the diatomic structure plotted for incident light in (i) forward and (j) backward directions.
Fig. 4. AT parameter dependence on the incident angle of light. AT parameter in the forward direction for a wide range of incident angles in (a) xz plane and (b) yz plane.
Fig. 5. Scattering power in terms of multipolar resonances. Calculated normalized multipolar decomposition for individual nanofins of the diatomic structure for (a), (b) RHCP and (c), (d) LHCP illumination in the forward direction. The red, blue, green, magenta, and black curves show the scattering power for the ED, MD, TD, EQ, and MQ modes, respectively.
Fig. 6. Electric and magnetic field distributions at different wavelengths in the visible regime. Calculated normalized EM field distributions at different wavelengths in the visible regime for the individual nanofins for RHCP and LHCP incident light. The normalized electric field distributions for the left and right nanofins at the wavelengths of 550, 640, and 700 nm for (a)–(f) RHCP and (g)–(i) LHCP illumination. Similarly, the normalized magnetic field distributions for the left and right nanofins at the wavelengths of 550, 640, and 700 nm for (m)–(r) RHCP and (s)–(x) LHCP illumination. The blue lines with white arrows show the direction of electric currents.
References | Wavelength (nm) | Design Material | Design Geometry | Broadband/Multiband | 3D/2D (Multilayer/Single layer) | Maximum AT | Maximum CD |
---|
[62] | 7000–12,000 | Silicon | Nanofins | Broadband | Single layer | at 9.6 μm | — | [63] | 540 | Titanium dioxide | Gammadation | Single band | Single layer | 0.9 | 0.8 | [64] | 550 | Silicon | Nanocube dimers | Single band | Single layer | — | CD enhancement up to 15-fold | [61] | 600 | Silver/silicon | Nanodisk | Single band | Single layer | — | CD enhancement in silicon | [57] | 1100–1600 | Silicon | Nanofins | Broadband | Bilayer | — | 0.7 at 1500 nm | [34] | 1655 | Silicon | z-shaped | Single band | Single layer | | — | [66] | 500–800 | a-Si:H | Nanofilms-based supercell | Broadband | Single layer | 0.58 | 0.55 | [67] | 550–700 | a-Si:H | Nanofin dimers | Broadband | Single layer | — | at 633 nm | This work | 550–700 | a-Si:H | Nanofins | Broadband | Single layer | | for FPa, exceeding for BPb |
|
Table 1. Comparison of the Relevant Literature of All-Dielectric Structures with the Proposed Design