
- Photonics Research
- Vol. 10, Issue 8, 1956 (2022)
Abstract
1. INTRODUCTION
Compared to the photodiode (PD), the avalanche photodiode (APD) can achieve higher sensitivity and quantum efficiency because of the internal gain mechanism. APD is widely used in optical sensing, quantum communication, LiDAR, and autonomous driving [1,2].
The III-V material-based APD suffers from high material cost and incompatibility with CMOS integration. These issues can be avoided by using germanium-on-silicon (Ge-on-Si) APDs. Being a small bandgap material, Ge has a strong linear absorption characteristic up to 1550 nm. The measured absorption coefficient is
Meanwhile, the waveguide coupling method has been widely adopted to improve the responsivity of Ge-on-Si APDs [6–8]. Compared with the traditional surface illumination detector [9–11], the waveguide integrated APD has a much longer absorption length to achieve a higher response rate. However, this type of micro-nano optical structure is unable to collect the scattered light signal in the field of free space communication efficiently, rendering it unsuitable for the application of unmanned and LiDAR detection.
Sign up for Photonics Research TOC. Get the latest issue of Photonics Research delivered right to you!Sign up now
In this work, a three-terminal surface illuminated Ge-on-Si APD is proposed. Previously, Zheng
2. DEVICE OPERATION MECHANISM, DESIGN, AND FABRICATION
Traditional Ge-on-Si APDs generally require large electric fields to obtain large gains in the avalanche regions. The large electric field can easily deplete the charge layer and then extend into the Ge absorption layer. But there is a mass of dislocations in the epitaxial Ge absorption layer on Si due to the lattice mismatches at the interface. These dislocations can form thermal generation-recombination centers and, thus, induce a large dark current under a large electric field [15]. In order to solve this problem, we proposed a three-terminal Ge-on-Si APD structure, as shown in Fig. 1(a). It consists of two parts. One part provides a large gain, and it is a traditional two-electrode Si-APD structure [as shown on the right side of Fig. 1(a)]. This part of the structure has the excellent performance of Si-APD, low dark current and high gain, but this structure cannot respond to the near-infrared light. Therefore, the other part of the structure provides photoinduced charge, which is in the Ge absorption region. The Ge region can generate photogenerated carriers for near-infrared light, and this absorption region is controlled by an independent electrode. The novelty of the APD in this work is that the Ge absorption region and traditional two-electrode Si-APD structure are connected through an extended Si charge region as a bridge. This region separates the absorption region from the Si-APD region. The electric field in the Ge absorption region is mainly determined by the voltage on the Ge electrode, while the electric field in the multiplication region of Si is controlled by the voltage applied between
Figure 1.(a) Structural diagram of three-terminal Ge-on-Si APD. (b) SEM image of pixel units. (c) Electric field simulation diagram of Ge region. (d) Simulated electric field in Si APD area [electric field at different positions of line A in (b)].
The devices were built on a silicon-on-insulator (SOI) wafer with 220 nm top-silicon, fabricated using the standard process in Advanced Micro Foundry (AMF), Singapore. Ge is grown by reduced pressure CVD (RPCVD), via selective Si epi growth. After the device is done, all the APDs are covered by oxide, which will help reduce the surface leakage current. The specific preparation steps of the device are as follows. The top-silicon layer was etched to define Si mesa patterns as shown in Fig. 1(b). Boron and phosphorus were implanted on the wafers to form p-type and n-type regions, respectively. The
Simulation was performed to examine the electric field in the device using TCAD software. Because the structure is three-dimensional and cannot be simplified in both the horizontal and vertical directions, three-dimensional device simulation is used. Figure 1(c) shows the simulated electric field in the germanium region. Figure 1(d) shows the electric field between the two electrodes of
3. DEVICE CHARACTERIZATION
A. Two-Terminal
The APD was characterized using the Keysight N7714A laser as the light source. Light was illuminated directly above the Ge active layer of the detectors through single mode fiber. Meanwhile, the probes contacted the APD’s electrode pads, which are connected to the Keysight 4200-SCS for data reading.
Due to the complexity of three-terminal APD, the device can be used as a traditional APD by floating one of the electrodes. The first configuration is to float the electrode on Ge and make the APD region in Si reversely biased. The observed light response is very weak because photo carriers in Ge cannot be swept into the APD region in Si to form a photocurrent. The second configuration is to apply reverse bias between Ge and the
Figure 2.(a) Current characteristic diagram at 1550 nm under different input optical power. (b) Responsivity characteristic diagram at 1550 nm under different input optical power. (c) Current characteristic diagram at 1310 nm under different input optical power. (d) Responsivity characteristic diagram at 1310 nm under different input optical power. The figure shows the voltage applied to two terminals Ge and
B. Three-Terminal
To better understand the device physics of three-terminal APD, three-dimensional simulation with TCAD software was performed. A fixed voltage is applied on the Ge electrode, and the
The trend of the simulated current is consistent with the measured results as shown in Figs. 3 and 4(a), and the electric field from TCAD software simulation is depicted in Fig. 5. Figure 5(b) shows the electric field in Si along the
Figure 3.It represents the simulated dark current diagram (current output at the
Figure 4.When the
Figure 5.(a) Schematic diagram of three-terminal APD. (b)
Due to the existence of the bridge region [see Fig. 5(a)], the Ge absorption region and the Si multiplication region are separated. As shown in Fig. 5(c), we can see that
Dark current at different voltages on Ge was measured, as shown in Fig. 4(a). As expected, the dark current increases with the increase of the applied voltage on the Ge electrode. The difference in breakdown voltage (defined as the voltage when dark current is
Figure 6.(a) Photocurrent diagram at
Figure 7.When the voltage on Ge is
We define the responsivity of the
Response of Ge to −27.5 V at Different Input Power
0.80 A/W | 3.16 A/W | 6.17 A/W | 10.50 A/W | |
0.63 A/W | 1.80 A/W | 1.82 A/W | 1.74 A/W | |
0.83 A/W | 3.55 A/W | 6.96 A/W | 9.97 A/W | |
0.68 A/W | 2.36 A/W | 2.48 A/W | 2.07 A/W |
When the voltage on Ge is
Figure 8.When the voltage on Ge is
Figure 9.When the applied voltage on
In order to verify the good detection characteristics of the device under weak light condition, we have compared the light and dark current of 1550 nm laser when the input power is
Figure 10.(a) Current at
4. DEVICE EQUIVALENT CIRCUIT MODEL
The electric circuit model of a three-terminal Ge-on-Si APD is much more complex than that of a conventional two-terminal APD [23] because multiple current paths exist between any two of the three electric terminals. In order to analyze the bandwidth property of this APD, radio frequency (RF) response characteristics from 10 to 600 MHz of it are measured using the Keysight N4373D Lightwave Component Analyzer. To simplify the measurement, we used a GS probe to load on the electrodes of
Figure 11.(a) The APD has a normalized RF response characteristic at a voltage of Ge at
Figure 12.(a) When the voltage is
5. CONCLUSION
We propose a new Ge-on-Si APD structure, which uses the extended p-charge layer to separate the Ge absorption and Si-APD region. We use Si material with low-noise properties as the multiplication region to reduce the excess noise of the APD [33]. By introducing three electrodes to control the electric fields in the absorption and avalanche region, respectively, the goals of low dark current and high responsivity of the surface-illuminated detector are achieved. Before us, the responsivity of surface illumination devices was very low, and high responsivity could only be achieved with a large dark current. We widened the limit of weak light detection to
Device Performance of Surface Illuminated APDs
Reference | Dark Current (A) | Responsivity (A/W) | |
---|---|---|---|
[ | 1310 | 6.54 | |
[ | 1310 | 12.00 | |
[ | 1550 | 12.70 | |
[ | 1310 | 4.40 | |
[ | 1310 | 5.88 | |
This work | 1310 | 10.50 | |
This work | 1550 | 9.97 |
This new type of APD can be manufactured with a high yield in a standard CMOS process. The different performance indicators of this APD, such as breakdown voltage, can be realized on the same wafer by design. The three-terminal APD has broken the inherent barrier of the single output of the previous APD device. This is the first and a major breakthrough.
References
[1] J. Zhang, M. A. Itzler, H. Zbinden, J. W. Pan. Advances in InGaAs/InP single-photon detector systems for quantum communication. Light Sci. Appl., 4, e286(2015).
[2] M. Wanitzek, M. Oehme, D. Schwarz, K. Guguieva, J. Schulze. Ge-on-Si avalanche photodiodes for LIDAR applications. 43rd International Convention on Information, Communication and Electronic Technology (MIPRO), 8-12(2020).
[3] V. Sorianello, A. Perna, L. Colace, G. Assanto, H. C. Luan, L. C. Kimerling. Near-infrared absorption of germanium thin films on silicon. Appl. Phys. Lett., 93, 111115(2008).
[4] Y. Ishikawa, K. Wada, D. D. Cannon, J. Liu, H.-C. Luan, L. C. Kimerling. Strain-induced band gap shrinkage in Ge grown on Si substrate. Appl. Phys. Lett., 82, 2044-2046(2003).
[5] J. M. Hartmann, A. Abbadie, A. M. Papon, P. Holliger, G. Rolland, T. Billon, J. M. Fédéli, M. Rouvière, L. Vivien, S. Laval. Reduced pressure-chemical vapor deposition of Ge thick layers on Si(001) for 1.3–1.55-μm photodetection. J. Appl. Phys., 95, 5905-5913(2004).
[6] H. T. Chen, J. Verbist, P. Verheyen, P. De Heyn, G. Lepage, J. De Coster, P. Absil, B. Moeneclaey, X. Yin, J. Bauwelinck, J. Van Campenhout, G. Roelkens. 25-Gb/s 1310-nm optical receiver based on a sub-5-V waveguide-coupled germanium avalanche photodiode. IEEE Photon. J., 7, 7902909(2015).
[7] A. Palmieri, M. Vallone, M. Calciati, A. Tibaldi, F. Bertazzi, G. Ghione, M. Goano. Heterostructure modeling considerations for Ge-on-Si waveguide photodetectors. Opt. Quantum Electron., 50, 71(2018).
[8] Z. Huang, C. Li, D. Liang, K. Yu, C. Santori, M. Fiorentino, W. Sorin, S. Palermo, R. G. Beausoleil. 25 Gbps low-voltage waveguide Si–Ge avalanche photodiode. Optica, 3, 793-798(2016).
[9] W. S. Zaoui, H.-W. Chen, J. E. Bowers, Y. Kang, M. Morse, M. J. Paniccia, A. Pauchard, J. C. Campbell. Frequency response and bandwidth enhancement in Ge/Si avalanche photodiodes with over 840 GHz gain-bandwidth-product. Opt. Express, 17, 12641-12649(2009).
[10] Y. Kang, M. Zadka, S. Litski, G. Sarid, M. Morse, M. J. Paniccia, Y. H. Kuo, J. Bowers, A. Beling, H. D. Liu, D. C. McIntosh, J. Campbell, A. Pauchard. Epitaxially-grown Ge/Si avalanche photodiodes for 1.3 μm light detection. Opt. Express, 16, 9365-9371(2008).
[11] G. Kim, S. Kim, S. A. Kim, J. H. Oh, K.-S. Jang. NDR-effect vertical-illumination-type Ge-on-Si avalanche photodetector. Opt. Lett., 43, 5583-5586(2018).
[12] X. Zeng, Z. Huang, B. Wang, D. Liang, M. Fiorentino, R. G. Beausoleil. Silicon-germanium avalanche photodiodes with direct control of electric field in charge multiplication region. Optica, 6, 772-777(2019).
[13] B. Wang, Z. Huang, X. Zeng, D. Liang, M. Fiorentino, R. G. Beausoleil. 35 Gb/s ultralow-voltage three-terminal Si-Ge avalanche photodiode. Optical Fiber Communications Conference and Exhibition (OFC), Th3B.2(2019).
[14] X. Zeng, Z. Huang, D. Liang, M. Fiorentino, R. G. Beausoleil. Low-voltage three-terminal avalanche photodiodes. Conference on Lasers and Electro-Optics, SF2I.3(2017).
[15] D. Benedikovic, L. Virot, G. Aubin, J.-M. Hartmann, F. Amar, X. Le Roux, C. Alonso-Ramos, É. Cassan, D. Marris-Morini, J.-M. Fédéli, F. Boeuf, B. Szelag, L. Vivien. Silicon-germanium receivers for short-wave-infrared optoelectronics and communications high-speed silicon-germanium receivers. Nanophotonics, 10, 1059-1079(2021).
[16] Z. Huang, X. Zeng, D. Liang, M. Fiorentino, R. G. Beausoleil. Operation and analysis of low-voltage three-terminal avalanche photodiodes. IEEE 14th International Conference on Group IV Photonics (GFP), 174-175(2017).
[17] L. Virot, P. Crozat, J.-M. Fédéli, J.-M. Hartmann, D. Marris-Morini, E. Cassan, F. Boeuf, L. Vivien. Germanium avalanche receiver for low power interconnects. Nat. Commun., 5, 4957(2014).
[18] Y. Li, X. Luo, G. Liang, G.-Q. Lo. Demonstration of Ge/Si avalanche photodetector arrays for lidar application. Optical Fiber Communication Conference, TU3E.3(2019).
[19] M. Huang, S. Li, P. Cai, G. Hou, T.-I. Su, W. Chen, C.-Y. Hong, D. Pan. Germanium on silicon avalanche photodiode. IEEE J. Sel. Top. Quantum Electron., 24, 3800911(2018).
[20] K. He, J. Lu, X. Ma, Y. Ju, L. Xie, L. Pang, X. Wang, J. Chen. Effect of Maxwell–Wagner relaxation on field charging of particles. Aerosol Sci. Technol., 49, 1210-1221(2015).
[21] D. Dai, M. Piels, J. E. Bowers. Monolithic germanium/silicon photodetectors with decoupled structures: resonant APDs and UTC photodiodes. IEEE J. Sel. Top. Quantum Electron., 20, 3802214(2014).
[22] H.-Y. Zhao, , A. H. Jones, R.-L. Chao, Z. Ahmad, J. C. Campbell, J.-W. Shi. High-speed avalanche photodiodes with wide dynamic range performance. J. Lightwave Technol., 37, 5945-5952(2019).
[23] B. Wang, Z. Huang, X. Zeng, R. Wu, W. V. Sorin, D. Liang, R. G. Beausoleil. A compact model for Si-Ge avalanche photodiodes. IEEE 15th International Conference on Group IV Photonics (GFP), 109-110(2018).
[24] D. R. Gozzard, L. E. Roberts, J. T. Spollard, P. G. Sibley, D. A. Shaddock. Fast beam steering with an optical phased array. Opt. Lett., 45, 3793-3796(2020).
[25] K. Van Acoleyen, K. Komorowska, W. Bogaerts, R. Baets. One-dimensional off-chip beam steering and shaping using optical phased arrays on silicon-on-insulator. J. Lightwave Technol., 29, 3500-3505(2011).
[26] D. Kwong, A. Hosseini, Y. Zhang, R. T. Chen. 1 × 12 unequally spaced waveguide array for actively tuned optical phased array on a silicon nanomembrane. Appl. Phys. Lett., 99, 051104(2011).
[27] D. Dai, M. J. W. Rodwell, J. E. Bowers, Y. Kang, M. Morse. Derivation of the small signal response and equivalent circuit model for a separate absorption and multiplication layer avalanche photodetector. IEEE J. Sel. Top. Quantum Electron., 16, 1328-1336(2010).
[28] J.-M. Lee, S.-H. Cho, W.-Y. Choi. An equivalent circuit model for a Ge waveguide photodetector on Si. IEEE Photon. Technol. Lett., 28, 2435-2438(2016).
[29] B. Wang, Z. Huang, X. Zeng, W. V. Sorin, D. Liang, M. Fiorentino, R. G. Beausoleil. A compact model for Si-Ge avalanche photodiodes over a wide range of multiplication gain. J. Lightwave Technol., 37, 3229-3235(2019).
[30] D. Dai, H.-W. Chen, J. E. Bowers, Y. Kang, M. Morse, M. J. Paniccia. Equivalent circuit model of a Ge/Si avalanche photodiode. 6th IEEE International Conference on Group IV Photonics, 13-15(2009).
[31] M.-J. Lee, H.-S. Kang, W.-Y. Choi. Equivalent circuit model for Si avalanche photodetectors fabricated in standard CMOS process. IEEE Electron Device Lett., 29, 1115-1117(2008).
[32] D. Dai, H.-W. Chen, J. E. Bowers, Y. Kang, M. Morse, M. J. Paniccia. Resonant normal-incidence separate-absorption-charge-multiplication Ge/Si avalanche photodiodes. Opt. Express, 17, 16549-16557(2009).
[33] Y. Kang, H.-D. Liu, M. Morse, M. J. Paniccia, M. Zadka, S. Litski, G. Sarid, A. Pauchard, Y.-H. Kuo, H.-W. Chen, W. S. Zaoui, J. E. Bowers, A. Beling, D. C. McIntosh, X. Zheng, J. C. Campbell. Monolithic germanium silicon avalanche photodiodes with 340 GHz gain-bandwidth product. Nat. Photonics, 3, 59-63(2009).
[34] B. Shi, F. Qi, P. Cai, X. Chen, Z. He, Y. Duan, G. Hou, T. Su, S. Li, W. Chen, C. Hong, R.-C. Yu, D. Pan. 106 Gb/s normal-incidence Ge/Si avalanche photodiode with high sensitivity. Optical Fiber Communication Conference, M3D.2(2020).
[35] C.-L. Hsin, C.-H. Chou. Buffer-free Ge/Si by rapid melting growth technique for separate absorption and multiplication avalanche photodetectors. IEEE Electron Device Lett., 40, 945-948(2019).
[36] M. Huang, T. Shi, P. Cai, L. Wang, S. Li, W. Chen, C.-Y. Hong, D. Pan. 25 Gb/s normal incident Ge/Si avalanche photodiode. Systematic Paris Region Systems and ICT Cluster, 1-3(2014).
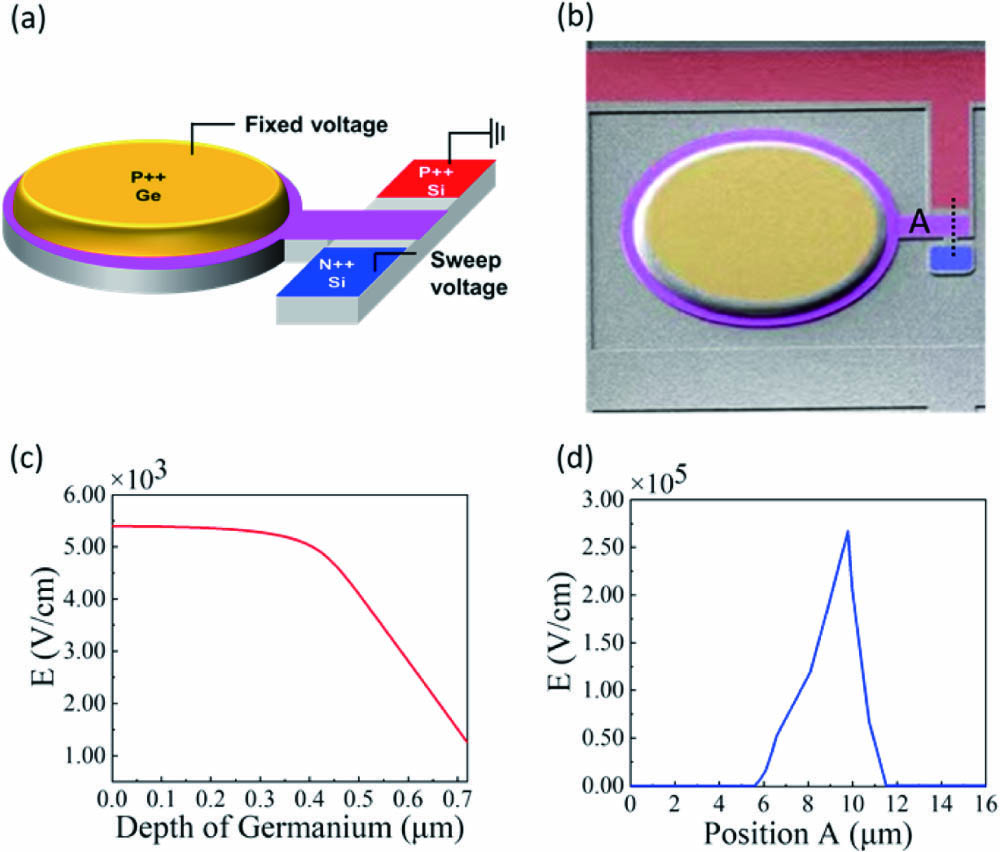
Set citation alerts for the article
Please enter your email address