A novel thin-film lithium niobate (TFLN) electro-optic modulator is proposed and demonstrated.

- Chinese Optics Letters
- Vol. 19, Issue 6, 060016 (2021)
Abstract
1. Introduction
High-speed electro-optic modulators are key devices for high-capacity fiber-optic communications[
Recently, thin-film LN (TFLN) fabricated by crystal ion slicing and wafer bonding[
For many applications, it is desirable to further reduce the half-wave-voltage length product
Sign up for Chinese Optics Letters TOC. Get the latest issue of Chinese Optics Letters delivered right to you!Sign up now
To break these performance limitations, we propose a TFLN modulator based on an LN-silica hybrid waveguide, which allows an electrode gap as narrow as 3 µm for enhanced electric field loading efficiency. Meanwhile, capacitively loaded traveling-wave electrodes (CL-TWEs) are adopted to reduce the microwave loss. Furthermore, to overcome the slow wave effect of the CL-TWEs, a quartz substrate with a low dielectric constant is employed to implement velocity matching between the microwave and the optical signals[
2. Design and Simulation
Figure 1(a) depicts the ridge waveguide structure commonly adopted in a TFLN modulator. The refractive index variation in the X-cut LN waveguide is given by[
Figure 1.(a) Conventional TFLN waveguide with wide-gap electrodes. (b) LN-silica hybrid waveguide with narrow-gap electrodes. (c) Optical absorption loss of optical waveguides with/without the silica buffer layer. RF modes in (d) TFLN waveguide with wide-gap electrodes and (e) LN-silica hybrid waveguide with narrow-gap electrodes.
Here,
The thickness of the silica buffer layer is optimized for a trade-off between a small electrode spacing and a large electro-optic overlap factor. Assuming an upper-limit of 0.1 dB/cm for optical absorption loss, the minimum allowable electrode spacing and the corresponding electro-optic overlap factor calculated by the finite element method (FEM) are plotted in Fig. 2(a). It is evident that the minimum electrode spacing reduces rapidly as the silica buffer layer thickness increases, whereas the electro-optic overlap factor shows only a moderate reduction. The refractive index variation in the LN-silica hybrid waveguide under 1 V drive voltage is shown in Fig. 2(b). A 100-nm-thick silica buffer layer with an electrode spacing of 3 µm is found to provide the optimum modulation efficiency.
Figure 2.(a) Minimum allowable electrode spacing and electro-optic overlap factor for different silica buffer layer thicknesses. (b) Refractive index variation of the hybrid waveguide with 1 V drive voltage. A 100-nm-thick silica layer is chosen to obtain the maximum refractive index change.
The key to extending the modulation bandwidth lies in reducing the microwave loss while implementing velocity and impedance matching[
Figure 3.(a) Top view of the CL-TWEs. (b) Duty cycle of T-rails for different T-rail gaps under capacitance matching condition. (c) Variation of microwave loss with the width of unloaded signal electrode under inductance matching condition. The star in (b) and (c) indicates the designed value.
The characteristic impedance and refractive index of the traveling-wave electrodes can be expressed as
3. Fabrication and Measurement
The modulator is fabricated with a wafer of TFLN on quartz provided by NanoLN, which includes a 2-µm-thick
Figure 4.(a) Demonstrated modulator 3D schematic. The unloaded electrodes have 50 µm signal electrode width, 15 µm electrode spacing, and bent tapers to match with the microwave probes. The inset shows the SEM image of the 3-µm-spaced T-rails with 50 µm period and 90% duty cycle. (b) Insertion loss of 5-mm-long modulators with different electrode gaps. (c) Normalized optical transmission as a function of modulation voltage. (d) Microwave transmission S21 and reflection S11 of the traveling-wave electrodes as well as the electro-optic response of the TFLN modulator up to 67 GHz. (e) Extracted microwave refractive index, which shows excellent matching with the group index of the optical mode (ng ∼ 2.25).
The performance of the modulator is tested by end-butt coupling with two tapered fibers, and a polarization controller is used to ensure TE polarized incident light at 1550 nm. In Fig. 4(b), we plot the insertion loss of 5-mm-long modulators with T-rail gaps of 3 and 4 µm. Compared with the device without electrodes, it can be concluded that the excessive optical loss due to narrow electrode gaps is effectively suppressed by the silica buffer layer, which is in agreement with the estimation shown in Fig. 1(c). The total insertion loss is measured to be 17 dB for a 5-mm-long modulator, which mainly comes from the coupling loss, as the end facets of the modulator need further polishing, and the mode field mismatch between the fiber and the optical waveguide has not been optimized. The coupling efficiency can be significantly improved by employing an inverse taper[
A frequency response test system with a bandwidth up to 67 GHz is used to characterize the electro-optic response of the modulator. First, the microwave transmission
Figure 5.Extended electrical bandwidth measurement to 110 GHz.
4. Conclusion
In this work, we have proposed a TFLN modulator structure capable of both wide modulation bandwidth and low half-wave-voltage length product. Modulators equipped with 3 µm electrode spacing CL-TWEs are fabricated on TFLN wafer bonded to a quartz substrate. A half-wave-voltage length product as low as 1.7 V·cm has been demonstrated with an LN-silica hybrid waveguide. The electro-optic response of a device with 5 mm modulation length shows a merely less than 2 dB roll-off up to 67 GHz.
References
[1] E. L. Wooten, K. M. Kissa, A. Yi-Yan, E. J. Murphy, D. A. Lafaw, P. F. Hallemeier, D. Maack, D. V. Attanasio, D. J. Fritz, G. J. McBrien, D. E. Bossi. A review of lithium niobate modulators for fiber-optic communications systems. IEEE J. Sel. Top. Quantum Electron., 6, 69(2000).
[2] J. Capmany, D. Novak. Microwave photonics combines two worlds. Nat. Photon., 1, 319(2007).
[3] K. Noguchi, O. Mitomi, H. Miyazawa. Millimeter-wave Ti:LiNbO3 optical modulators. J. Lightwave Technol., 16, 615(1998).
[4] M. Bazzan, C. Sada. Optical waveguides in lithium niobate: recent developments and applications. Appl. Phys. Rev., 2, 040603(2015).
[5] X. Xiao, M. Li, L. Wang, D. Chen, Q. Yang, S. Yu. High speed silicon photonic modulators. Optical Fiber Communication Conference, Tu2H.1(2017).
[6] A. Khilo, C. M. Sorace, F. X. Kärtner. Broadband linearized silicon modulator. Opt. Express, 19, 4485(2011).
[7] J. Liu, G. Xu, F. Liu, I. Kityk, X. Liu, Z. Zhen. Recent advances in polymer electro-optic modulators. RSC Adv., 5, 15784(2015).
[8] H. Miura, F. Qiu, A. M. Spring, T. Kashino, T. Kikuchi, M. Ozawa, H. Nawata, K. Odoi, S. Yokoyama. High thermal stability 40 GHz electro-optic polymer modulators. Opt. Express, 25, 28643(2017).
[9] R. G. Walker. High-speed III-V semiconductor intensity modulators. IEEE J. Quantum Electron., 27, 654(1991).
[10] Y. Ogiso, Y. Hashizume, H. Tanobe, N. Nunoya, M. Ida, Y. Miyamoto, M. Ishikawa, J. Ozaki, Y. Ueda, H. Wakita, M. Nagatani, H. Yamazaki, M. Nakamura, T. Kobayashi, S. Kanazawa. 80-GHz bandwidth and 1.5-V Vπ InP-based IQ modulator. J. Lightwave Technol., 38, 249(2020).
[11] G. Poberaj, H. Hu, W. Sohler, P. Günter. Lithium niobate on insulator (LNOI) for micro-photonic devices. Laser Photon. Rev., 6, 488(2012).
[12] C. Wang, M. Zhang, X. Chen, M. Bertrand, A. Shams-Ansari, S. Chandrasekhar, P. Winzer, M. Lončar. Integrated lithium niobate electro-optic modulators operating at CMOS-compatible voltages. Nature, 562, 101(2018).
[13] M. He, M. Xu, Y. Ren, J. Jian, Z. Ruan, Y. Xu, S. Gao, S. Sun, X. Wen, L. Zhou, L. Liu, C. Guo, H. Chen, S. Yu, L. Liu, X. Cai. High-performance hybrid silicon and lithium niobate Mach–Zehnder modulators for 100 Gbit·s−1 and beyond. Nat. Photon., 13, 359(2019).
[14] X. Wang, P. O. Weigel, J. Zhao, M. Ruesing, S. Mookherjea. Achieving beyond-100-GHz large-signal modulation bandwidth in hybrid silicon photonics Mach Zehnder modulators using thin film lithium niobate. APL Photon., 4, 096101(2019).
[15] A. N. R. Ahmed, S. Nelan, S. Shi, P. Yao, A. Mercante, D. W. Prather. Subvolt electro-optical modulator on thin-film lithium niobate and silicon nitride hybrid platform. Opt. Lett., 45, 1112(2020).
[16] S. Sun, M. He, M. Xu, S. Gao, Z. Chen, X. Zhang, Z. Ruan, X. Wu, L. Zhou, L. Liu, C. Lu, C. Guo, L. Liu, S. Yu, X. Cai. Bias-drift-free Mach–Zehnder modulators based on a heterogeneous silicon and lithium niobate platform. Photon. Res., 8, 1958(2020).
[17] A. Honardoost, F. A. Juneghani, R. Safian, S. Fathpour. Towards subterahertz bandwidth ultracompact lithium niobate electrooptic modulators. Opt. Express, 27, 6495(2019).
[18] X. Liu, B. Xiong, C. Sun, Z. Hao, L. Wang, J. Wang, Y. Han, H. Li, J. Yu, Y. Luo. Low half-wave-voltage thin film LiNbO3 electro-optic modulator based on a compact electrode structure. Asia Communications and Photonics Conference (ACP/IPOC 2020), M4A.144(2020).
[19] X. Liu, B. Xiong, C. Sun, Z. Hao, L. Wang, J. Wang, Y. Han, H. Li, Y. Luo. Thin film LiNbO3 modulator based on LiNbO3-silica hybrid waveguide with narrow electrode gap. 2020 International Topical Meeting on Microwave Photonics (MWP), 166(2020).
[20] Y.-K. Wu, W.-S. Wang. Design and fabrication of sidewalls-extended electrode configuration for ridged lithium niobate electrooptical modulator. J. Lightwave Technol., 26, 286(2008).
[21] M. Jin, J. Chen, Y. Sua, P. Kumar, Y. Huang. Efficient electro-optical modulation on thin-film lithium niobate. Opt. Lett., 46, 1884(2021).
[22] X. Zhang, T. Miyoshi. Optimum design of coplanar waveguide for LiNbO3 optical modulator. IEEE Trans. Microwave Theory Tech., 43, 523(1995).
[23] P. Kharel, C. Reimer, K. Luke, L. He, M. Zhang. Breaking voltage–bandwidth limits in integrated lithium niobate modulators using micro-structured electrodes. Optica, 8, 357(2021).
[24] X. Liu, B. Xiong, C. Sun, Z. Hao, L. Wang, J. Wang, Y. Han, H. Li, J. Yu, Y. Luo. Thin film lithium niobate electro-optic modulator based on a slow wave structure. 14th Pacific Rim Conference on Lasers and Electro-Optics (CLEO PR 2020), C4C_3(2020).
[25] J. Shin, S. R. Sakamoto, N. Dagli. Conductor loss of capacitively loaded slow wave electrodes for high-speed photonic devices. J. Lightwave Technol., 29, 48(2011).
[26] L. He, M. Zhang, A. Shams-Ansari, R. Zhu, C. Wang, L. Marko. Low-loss fiber-to-chip interface for lithium niobate photonic integrated circuits. Opt. Lett., 44, 2314(2019).
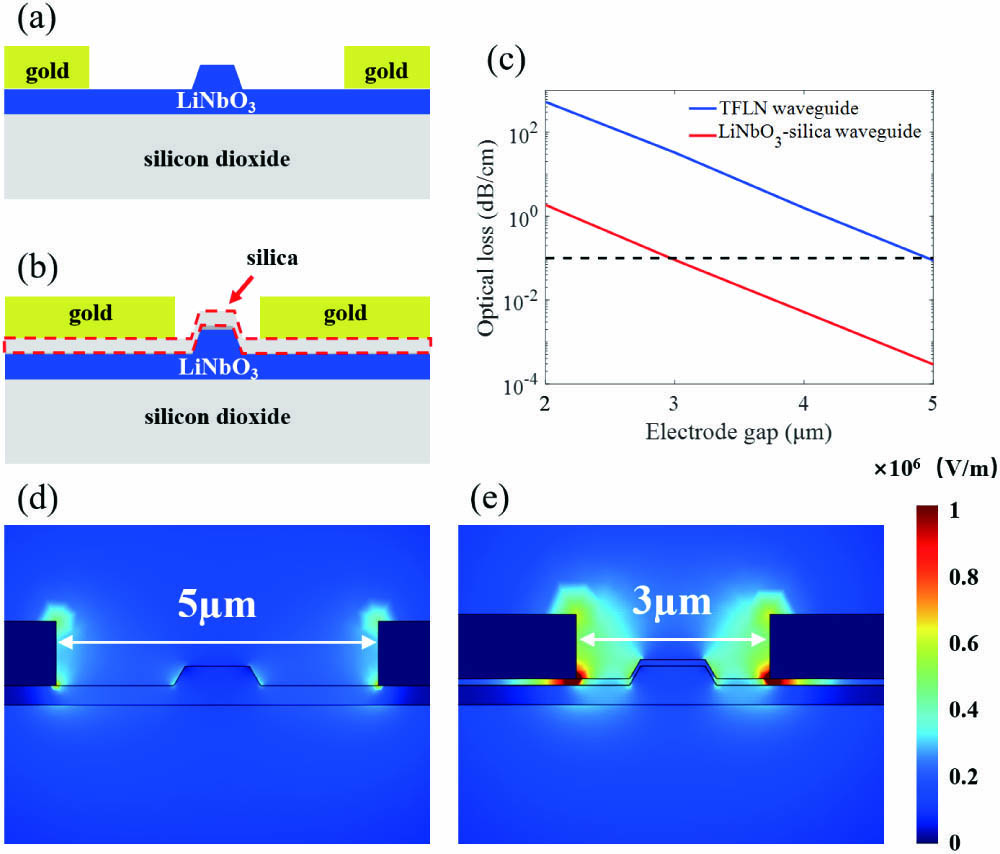
Set citation alerts for the article
Please enter your email address