
- Photonics Research
- Vol. 10, Issue 3, 646 (2022)
Abstract
1. INTRODUCTION
Simulation, computation, and number theory may have a demanding requirement for the generation rate of the exploited random numbers following the right statistics, while other applications, such as the classical communications based on the RSA cryptosystem, the current quantum key distribution [1,2], signature schemes [3,4], and quantum secret sharing [5], place greater demand on the security or privacy of random numbers. It has been reported that thousands of servers are attacked by inferior hackers for the lousy randomness of pseudo-random numbers every year [6]. It can be argued that the private randomness is a potential necessary condition of any secure and secret communications. Even in daily life, the security and the non-repeatability of random numbers can also be crucially significant. Defective products can pass the quality inspection exploiting random sampling just because the illegitimate manufacturer gets the random numbers for sampling and changes the order of products beforehand. An illegal gambler may get a bigger bang for the buck by receiving partial random numbers and adopting the best guessing strategy. A lottery draw may be rigged by an unlawful manager, who obtains the side information of the random number generator during routine maintenance.
Leaning toward the mainstream viewpoint, random numbers generated by classical systems are all pseudo-random numbers, while only quantum theory can offer true randomness [7,8]. The earliest quantum random number generator (QRNG) is based on radioactive decay [9], which generates true random numbers with a low bit rate taking demanding safety measures. Moreover, the source generating quantum random numbers (i.e., entropy source) can be quantum tunneling [10,11], Majorana fermions [12], quantum fluctuations of the collective spin of an alkali-metal vapor [13], phase noise of a single-mode laser [14], etc. However, there have been demonstrations of random numbers generated on a mobile phone [15], in which the random numbers are generated from resolving photon-number distribution of a camera. Most QRNGs are currently based on quantum optics since different quantum states with inherent randomness offer a rich choice of implementations and complete analytical methods [7,8,16–25]. The polarization of single photons [26], temporal [27, 28] or spatial mode [29,30] of photons can generate random bits; photon counting [31], amplified spontaneous emission [32,33], and stimulated Raman scattering [34] can also be the entropy source. In recent years, there have been reports of a quantum random number generator with a photonic integrated chip [21] and even a quantum random number cloud platform [35]. Among all quantum resources, measurement of the vacuum noise via homodyne detection is one of the most efficient and economical methods with high-speed and secure bits generation [36–38]. For a general entropy source, it is far from trivial to estimate the min-entropy. Consequently, there have been many QRNG protocols [7,8]. In practical terms the measurement device can be characterized easily by the user at close range, while the entropy source and local oscillator (LO) can be distributed remotely for the convenience of users. Therefore, the entropy source and LO are more vulnerable to attack. Based on this consideration, the QRNG protocol with an untrusted source and indeterminate LO is needed, i.e., the source-device-independent (SDI) QRNG. In the SDI QRNG, the secure randomness needs to be estimated and quantified in the presence of the eavesdropper attacking the source. Finally, the private random bits can be extracted by the Toeplitz-matrix hashing algorithm from the raw random numbers.
The entropic uncertainty principle (EUP) [39,40] gives a lower bound on the conditional min-entropy that estimates the amount of secure randomness in the presence of the eavesdropper and is used to calibrate the randomness extractor in the SDI QRNG where the entropy source is completely untrusted [41–43]. A pair of non-commutative operators
Sign up for Photonics Research TOC. Get the latest issue of Photonics Research delivered right to you!Sign up now
In fact, the check quadrature
The implementation of our protocol is shown in Fig. 1. An untrusted coherent state (CS) is divided into two identical parts (CS1 and CS2 with equal power and fluctuation) in which quadrature
Figure 1.Flow diagram of the experimental structure. An untrusted coherent state (CS) is divided into two identical and probably impure parts, CS1 for measuring quadrature
When an untrusted state is used as the entropy source, the amount of private random bits based on the EUP is given by [40,41,46–51]
In fact, the coherent state before division is an uncharacterized state in our mutually testing QRNG protocol. Therefore, the total amount of extractable randomness of two separated impure states (CS1 and CS2, in Fig. 1) can be represented as
Taking into account the finite-size effects and the security of the extractor during the randomness extraction, the bound on the conditional min-entropy should be further lowered as
It is a remarkable fact that the electronic noise may be controlled by the eavesdropper, so it needs to be measured at random times. Moreover, the fluctuations of the LO need to be monitored since the fluctuations can add insecure noise to the measured noise of the quadratures via an imperfect homodyne detector from the eavesdropper [53]. The collected data consist of the vacuum noise, extra noise introduced by LO fluctuations, and electronic noise of the detection system:
2. PRINCIPLE AND EXPERIMENTAL SETUP
The schematic of the experimental setup of the mutually testing SDI QRNG is illustrated in Fig. 2. A laser beam at the wavelength of 1342 nm from the
Figure 2.Experimental schematic configuration for mutually testing SDI QRNG. The pink area is a private space that no eavesdropper has access to. The black and blue curves represent the electric and data cables, respectively. The coherent state is generated via a laser and MC. The laser beam is divided into the signal beam and LO via a 98:2 BS. Both the signal beam and the LO are split in half via two 50:50 BSs. Two BHDs are used to measure the quadrature
3. RESULTS
In our experiment, the electronic noise is basically maintained because there is no interference in the surroundings of the detectors from the eavesdropper. The measured shot noises of two BHDs severally contain (
Figure 3.Red, blue, and black curves show the autocorrelations calculated from the raw bits, the downsampled bits, and the extracted bits, respectively. The three data streams have the same length of
4. CONCLUSION
In summary, we experimentally demonstrate a mutually testing SDI QRNG based on an untrusted source and a trusted equipartition process. The lower bounds on the amount of secure randomness of a pair of conjugate quadratures of two identical states are mutually estimated with the simultaneously measured data of the two quadratures; thus, it is convenient for users to avoid switching the measurement types of quadrature components. An SDI QRNG protocol based on the EUP is exploited to make the terminal random bits secure from the eavesdropper’s attacks on the entropy source. Moreover, the electronic noise of the homodyne detection system and the fluctuations of the LO are monitored to eliminate the interference around the detectors and resist the attacks on the LO to improve the total security. A coherent state is divided into two identical parts in which the quadrature
APPENDIX A: BETWEEN MUTUAL TESTING AND RANDOM TOGGLING
As mentioned above, the mutually testing SDI QRNG can generate private random numbers with a higher bit rate than randomly toggling QRNG. This point can be seen intuitively from the data acquisition and appropriate time sequences. The data acquisitions and appropriate time sequences for the mutually testing and randomly toggling QRNGs are shown schematically in Fig.
Figure 4.Comparison of the data acquisitions and appropriate time sequences of mutually testing and randomly toggling manners. The red and blue points represent the measured data of quadratures
APPENDIX B: MEASUREMENT OF QUADRATURES WITH HOMODYNE DETECTION
A schematic of the balanced homodyne detection is shown in Fig.
Figure 5.Schematic of the balanced homodyne detection. The difference current is converted into an amplified voltage signal by a transimpedance amplifier.
The output current of the detector is proportional to the number of detected photons. The currents can be expressed by
The difference current is
Assuming the LO to be strong enough and in the coherent state, the LO can be regarded as a classical field with a mean value
APPENDIX C: Supplement to entropic uncertainty principle and estimation of generation rate
For the measured noise of quadratures
Then the lower bound on the conditional min-entropy is given by
In addition, the measurement accuracies
The effective sampling rate of raw data is 1 GS/s, and the security parameter is set as
References
[1] H.-K. Lo, H. F. Chau. Unconditional security of quantum key distribution over arbitrarily long distances. Science, 283, 2050-2056(1999).
[2] S.-K. Liao, W.-K. Cai, W.-Y. Liu, L. Zhang, Y. Li, J.-G. Ren, J. Yin, Q. Shen, Y. Cao, Z.-P. Li, F.-Z. Li, X.-W. Chen, L.-H. Sun, J.-J. Jia, J.-C. Wu, X.-J. Jiang, J.-F. Wang, Y.-M. Huang, Q. Wang, Y.-L. Zhou, L. Deng, T. Xi, L. Ma, T. Hu, Q. Zhang, Y.-A. Chen, N.-L. Liu, X.-B. Wang, Z.-C. Zhu, C.-Y. Lu, R. Shu, C.-Z. Peng, J.-Y. Wang, J.-W. Pan. Satellite-to-ground quantum key distribution. Nature, 549, 43-47(2017).
[3] P. J. Clarke, R. J. Collins, V. Dunjko, E. Andersson, J. Jeffers, G. S. Buller. Experimental demonstration of quantum digital signatures using phase-encoded coherent states of light. Nat. Commun., 3, 1174(2012).
[4] R. J. Collins, R. J. Donaldson, V. Dunjko, P. Wallden, P. J. Clarke, E. Andersson, J. Jeffers, G. S. Buller. Realization of quantum digital signatures without the requirement of quantum memory. Phys. Rev. Lett., 113, 040502(2014).
[5] Y. Zhou, J. Yu, Z. Yan, X. Jia, J. Zhang, C. Xie, K. Peng. Quantum secret sharing among four players using multipartite bound entanglement of an optical field. Phys. Rev. Lett., 121, 150502(2018).
[6] N. Heninger, Z. Durumeric, E. Wustrow, J. A. Halderman. Mining your Ps and Qs: detection of widespread weak keys in network devices. Proceeding of the 21st USENIX Security Symposium, 205-220(2012).
[7] X. Ma, X. Yuan, Z. Cao, B. Qi, Z. Zhang. Quantum random number generation. npj Quantum Inf., 2, 16021(2016).
[8] M. Herrero-Collantes, J. C. Garcia-Escartin. Quantum random number generators. Rev. Mod. Phys., 89, 015004(2017).
[9] M. Isida, H. Ikeda. Random number generator. Ann. Inst. Stat. Math., 8, 119-126(1956).
[10] D. Vartsky, D. Bar, P. Gilad, A. Schon. High-speed, true random-number generator. U.S. patent(2011).
[11] K. Aungskunsiri, R. Amarit, K. Wongpanya. Random number generation from a quantum tunneling diode. Appl. Phys. Lett., 119, 074002(2021).
[12] D.-L. Deng, L.-M. Duan. Fault-tolerant quantum random-number generator certified by Majorana fermions. Phys. Rev. A, 88, 012323(2013).
[13] G. E. Katsoprinakis, M. Polis, A. Tavernarakis, A. T. Dellis, I. K. Kominis. Quantum random number generator based on spin noise. Phys. Rev. A, 77, 054101(2008).
[14] B. Qi, Y.-M. Chi, H.-K. Lo, L. Qian. High-speed quantum random number generation by measuring phase noise of a single-mode laser. Opt. Lett., 35, 312-314(2010).
[15] B. Sanguinetti, A. Martin, H. Zbinden, N. Gisin. Quantum random number generation on a mobile phone. Phys. Rev. X, 4, 031056(2014).
[16] J. Yang, J. Liu, Q. Su, Z. Li, F. Fan, B. Xu, H. Guo. 5.4 Gbps real time quantum random number generator with simple implementation. Opt. Express, 24, 27475-27481(2016).
[17] Y.-H. Li, X. Han, Y. Cao, X. Yuan, Z.-P. Li, J.-Y. Guan, J. Yin, Q. Zhang, X. Ma, C.-Z. Peng, J.-W. Pan. Quantum random number generation with uncharacterized laser and sunlight. njp Quantum Inf., 5, 97(2019).
[18] Y.-Q. Nie, L. Huang, Y. Liu, F. Payne, J. Zhang, J.-W. Pan. 68 Gbps quantum random number generation by measuring laser phase fluctuations. Rev. Sci. Instrum., 86, 063105(2015).
[19] S.-H. Sun, F. Xu. Experimental study of a quantum random-number generator based on two independent lasers. Phys. Rev. A, 96, 062314(2017).
[20] Q. Zhang, D. Kong, Y. Wang, H. Zou, H. Chang. Dual-entropy-source quantum random number generation based on spontaneous emission. Opt. Lett., 45, 304-307(2020).
[21] B. Bai, J. Huang, G.-R. Qiao, Y.-Q. Nie, W. Tang, T. Chu, J. Zhang, J.-W. Pan. 18.8 Gbps real-time quantum random number generator with a photonic integrated chip. Appl. Phys. Lett., 118, 264001(2021).
[22] M. Ren, E. Wu, Y. Liang. Quantum random-number generator based on a photon-number-resolving detector. Phys. Rev. A, 83, 023820(2011).
[23] Z. L. Yuan, M. Lucamarini, J. F. Dynes, B. Frohlich, A. Plews, A. J. Shields. Robust random number generation using steady-state emission of gain-switched laser diodes. Appl. Phys. Lett., 104, 261112(2014).
[24] C. Abellan, W. Amaya, D. Domenech, P. Munoz, J. Capmany, S. Longhi, M. W. Mitchell, V. Pruneri. Quantum entropy source on an InP photonic integrated circuit for random number generation. Optica, 3, 989-994(2016).
[25] Q. Zhou, R. Valivarthi, C. John, W. Tittel. Practical quantum random-number generation based on sampling vacuum fluctuations. Quantum Eng., 1, e8(2019).
[26] P. X. Wang, G. Longo, Y. S. Li. Scheme for a quantum random number generator. J. Appl. Phys., 100, 056107(2006).
[27] Y.-Q. Nie, H.-F. Zhang, Z. Zhang, J. Wang, X. Ma, J. Zhang, J.-W. Pan. Practical and fast quantum random number generation based on photon arrival time relative to external reference. App. Phys. Lett., 104, 051110(2014).
[28] H.-Q. Ma, Y. Xie, L.-A. Wu. Random number generation based on the time of arrival of single photons. Appl. Opt., 44, 7760-7763(2005).
[29] Q. Luo, Z. Cheng, J. Fan, L. Tan, H. Song, G. Deng, Y. Wang, Q. Zhou. Quantum random number generator based on single-photon emitter in gallium nitride. Opt. Lett., 45, 4224-4227(2020).
[30] Q. Yan, B. Zhao, Q. Liao, N. Zhou. Multi-bit quantum random number generation by measuring positions of arrival photons. Rev. Sci. Instrum., 85, 103116(2014).
[31] E. de Jesus Lopes Soares, F. A. Mendonça, R. V. Ramos. Quantum random number generator using only one single photon detector. IEEE Photonics Technol. Lett., 26, 851-853(2014).
[32] L. Li, A. Wang, P. Li, H. Xu, L. Wang, Y. Wang. Random bit generator using delayed self-difference of filtered amplified spontaneous emission. IEEE Photonics J., 6, 7500109(2014).
[33] C. R. S. Williams, J. C. Salevan, X. Li, R. Roy, T. Murphy. Fast physical random number generator using amplified spontaneous emission. Opt. Express, 18, 23584-23597(2010).
[34] P. J. Bustard, D. G. England, J. Nunn, D. Moffatt, M. Spanner, R. Lausten, B. J. Sussman. Quantum random bit generation using energy fluctuations in stimulated Raman scattering. Opt. Express, 21, 29350-29357(2013).
[35] L. Huang, H. Zhou, K. Feng, C. Xie. Quantum random number cloud platform. npj Quantum Inf., 7, 107(2021).
[36] J. Ma, A. Hakande, X. Yuan, X. Ma. Coherence as a resource for source-independent quantum random-number generation. Phys. Rev. A, 99, 022328(2019).
[37] Z. Zheng, Y. Zhang, W. Huang, S. Yu, H. Guo. 6 Gbps real-time optical quantum random number generator based on vacuum fluctuation. Rev. Sci. Instrum., 90, 043105(2019).
[38] M. Huang, Z. Chen, Y. Zhang, H. Guo. A Gaussian-distributed quantum random number generator using vacuum shot noise. Entropy, 22, 618(2020).
[39] C.-F. Li, J.-S. Xu, X.-Y. Xu, K. Li, G.-C. Guo. Experimental investigation of the entanglement-assisted entropic uncertainty principle. Nat. Phys., 7, 752-756(2011).
[40] F. Furrer, M. Berta, M. Tomamichel, V. B. Scholz, M. Christandl. Position-momentum uncertainty relations in the presence of quantum memory. J. Math. Phys., 55, 122205(2014).
[41] D. G. Marangon, G. Vallone, P. Villoresi. Source-device-independent ultra-fast quantum random number generation. Phys. Rev. Lett., 118, 060503(2017).
[42] M. Avesani, D. G. Marangon, G. Vallone, P. Villoresi. Source-device-independent heterodyne-based quantum random number generator at 17 Gbps. Nat. Commun., 9, 5365(2018).
[43] T. Michel, J. Y. Haw, D. G. Marangon, O. Thearle, G. Vallone, P. Villoresi, P. K. Lam, S. M. Assad. Real-time source independent quantum random number generator with squeezed states. Phys. Rev. Appl., 12, 034017(2019).
[44] Z. Cao, H. Zhou, X. Yuan, X. Ma. Source-independent quantum random number generation. Phys. Rev. X, 6, 011020(2016).
[45] G. Vallone, D. G. Marangon, M. Tomasin, P. Villoresi. Quantum randomness certified by the uncertainty principle. Phys. Rev. A, 90, 052327(2014).
[46] W. Beckner. Inequalities in Fourier analysis. Ann. Math., 102, 159-182(1975).
[47] I. Bialynicki-Birula, J. Mycielski. Uncertainty relations for information entropy in wave mechanics. Comm. Math. Phys., 44, 129-132(1975).
[48] R. Konig, R. Renner, C. Schaffner. The operational meaning of min- and max-entropy. IEEE Trans. Inf. Theory, 55, 4337-4347(2009).
[49] L. Rudnicki, S. P. Walborn, F. Toscano. Optimal uncertainty relations for extremely coarse-grained measurements. Phys. Rev. A, 85, 042115(2012).
[50] F. Furrer, J. Aberg, R. Renner. Min- and max-entropy in infinite dimensions. Math. Phys., 306, 165-186(2011).
[51] T. Eberle, V. Handchen, J. Duhme, T. Franz, F. Furrer, R. Schnabel, R. F. Werner. Gaussian entanglement for quantum key distribution from a single-mode squeezing source. New J. Phys., 15, 053049(2013).
[52] H. J. Landau, H. O. Pollak. Prolate spheroidal wave functions, Fourier analysis and uncertainty – ii. Bell Syst. Tech. J., 40, 65-84(1961).
[53] W. Huang, Y.-C. Zhang, Z. Zheng, Y. Li, B. Xu, S. Yu. Practical security analysis of a continuous-variable quantum random-number generator with a noisy local oscillator. Phys. Rev. A, 102, 012422(2020).
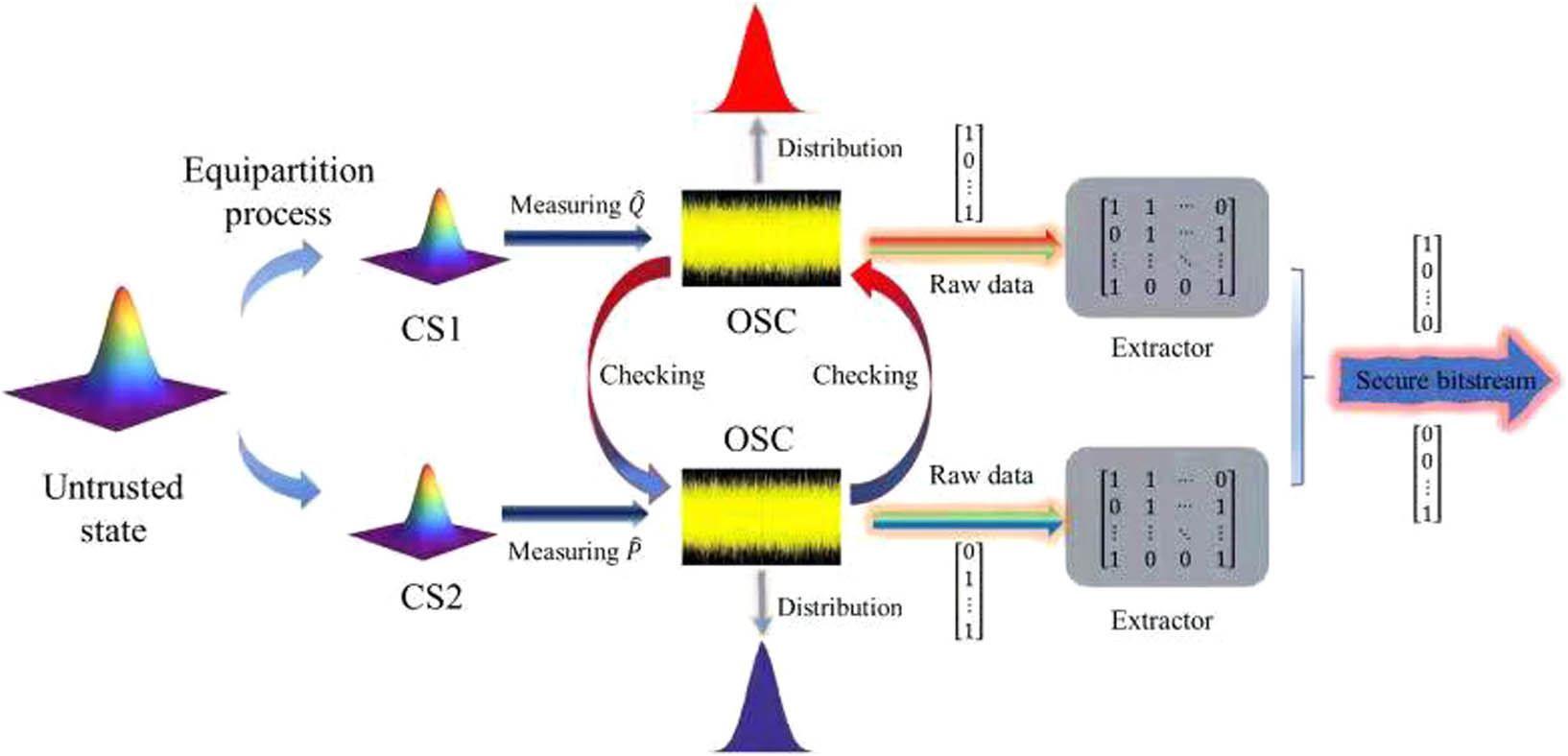
Set citation alerts for the article
Please enter your email address