
- Chinese Optics Letters
- Vol. 19, Issue 8, 081406 (2021)
Abstract
1. Introduction
Laser absorption spectroscopy is a powerful tool for precise quantification of the composition of solids[
The most common method of obtaining a mid-IR comb relies on frequency conversion of a near-IR mode-locked laser source by means of nonlinear techniques: optical parametric oscillator (OPO) and difference frequency generation (DFG). The OPOs can provide a multi-watt output combined with broad tunability[
Here, we present a robust, mid-IR comb source based on the DFG effect in a PPLN crystal, operating in the 3 µm range suitable for spectroscopy of hydrocarbons. The simple configuration requires a single EDFL as a seed source for both pump and signal generation. The seed laser and amplifiers are built all-in-fiber, so the setup is practically alignment-free. Moreover, active stabilization of crucial emission parameters [repetition frequency (
Sign up for Chinese Optics Letters TOC. Get the latest issue of Chinese Optics Letters delivered right to you!Sign up now
2. Experimental Results
The experimental setup is depicted in Fig. 1. The heart of the source is a graphene mode-locked EDFL operating at a center wavelength of 1565 nm, generating 320 fs pulses at a repetition rate of
Figure 1.Schematic of the fiber-based mid-IR comb source. PD, piezo driver; PID, proportional integral derivative controller; LO, local oscillator; Mix, RF mixer; FC, fiber coupler; EDFA/YDFA, Er- or Yb-doped fiber amplifier; HNLF, highly nonlinear fiber; DCF, dispersion compensating fiber; ODL, fiberized optical delay line; PZT, piezoceramic fiber stretcher; PMF, polarization-maintaining single-mode fiber; COLL, collimator; GC, grating compressor; FL, focusing lens; PPLN, 3-mm-long periodically poled lithium niobite crystal; G, germanium filter; BS, beam splitter; DET, MCT detector; AMP, RF amplifier; LA, logarithmic amplifier. Fibers are indicated in black, and electrical connections are in green.
Figure 2.Optical spectrum of the compressed 1565 nm pulses. The inset shows the measured autocorrelation trace.
In the second branch of the source (upper part of Fig. 1), the 1565 nm pulses are boosted to 280 mW of average power and a 55 fs duration before reaching an HNLF, in which a DW generation process occurs. Note that the HNLF is spliced to the amplifier output and therefore does not require any alignment. As a result, broadband radiation centered at 1060 nm is achieved. Next, the DW is boosted in a custom-built Yb-doped pre-amplifier and subsequently in a double-clad Yb-doped amplifier. The 1060 nm pulses are then directed to a transmissive grating-based compressor (GC). At the output, the pulses have a 195 fs duration and reach 1.9 W of average power. The optical spectrum of the compressed 1 µm pulses along with the autocorrelation measurement is depicted in Fig. 3.
Figure 3.Optical spectrum of the compressed 1 µm pulses. The inset shows the measured autocorrelation trace.
The pulses from the 1.56 µm branch and the 1 µm branch of the source are co-aligned on a dichroic mirror and directed through a 75 mm lens into a 3-mm-long PPLN crystal with five quasi-phase matching (QPM) periods (29.52–31.59 µm). Both branches of the source are designed and built so that their optical path lengths are equal. As a result of the nonlinear DFG process in the crystal, broadband radiation in the vicinity of 3.2 µm is generated. The emitted central wavelength can be tuned between
Figure 4.Generated optical spectra for different PPLN crystal periods, with average output power indicated in each measurement. Absorption lines of several molecules within the spectral coverage of the source are depicted in the upper panel.
In femtosecond DFG sources, crucial parameters of the emitted radiation, e.g., the average output power and the shape of the spectral envelope, strongly depend on the temporal overlap between the pulses interacting in the nonlinear medium. Therefore, active stabilization of the 1 µm and 1.56 µm pulse overlap is obligatory for long-term repeatability, required, e.g., in broadband gas absorption spectroscopy applications. To stabilize the temporal overlap, we use the method demonstrated in Refs. [25,26], in which an error signal is obtained from the measurement of the relative intensity noise (RIN) in the idler. As shown in the lower part of Fig. 1, a part of the generated mid-IR beam is directed to a mercury cadmium telluride (MCT) detector, whose output voltage signal is low-pass filtered (
The performance of the stabilization loop is depicted in Fig. 5. If no stabilization of the pulse overlap is employed, the spectral envelopes [Fig. 5(a)] and the average output power [Fig. 5(b)] fluctuate significantly during a 120 min period due to external perturbations (mostly temperature drift of the fibers). The active feedback loop used in our source compensates the thermal drift and ensures tight overlap of the pulses during long-term operation. After stabilizing the pulse overlap, the average output power fluctuation did not exceed a
Figure 5.(a) Heatmaps showing the time evolution of the spectrum for non-stabilized and stabilized cases. (b) Average output power stability as a function of time.
To verify the usability of the mid-IR comb source in gas spectroscopy applications, a proof-of-concept experiment was conducted, in which the broadband laser was used to detect absorption lines of
Figure 6.Absorption spectrum of CH4 and C2H6 measured using the DFG comb and a Fourier transform spectrometer (black) compared to the spectra of the two molecules (blue and red). The residuum of the fit is shown in the lower panel.
3. Conclusions
In this paper, a fully stabilized, tunable mid-IR DFG comb source is presented. The source is built using commercially available components in an all-PMF configuration and uses a single mode-locked EDFL as a seed. Tuning of the source parameters (temperature and QPM period of the crystal and the spectral position of the DW) enables covering the spectrum between
References
[1] W. M. Yen, P. M. Selzer. Laser Spectroscopy of Solids(2013).
[2] V. Lazic, S. Jovićević. Laser induced breakdown spectroscopy inside liquids: processes and analytical aspects. Spectrochim. Acta B At. Spectrosc., 101, 288(2014).
[3] Y. Ma, A. Vicet, K. Krzempek. State-of-the-art laser gas sensing technologies. Appl. Sci., 10, 433(2020).
[4] B. Fu, C. Zhang, W. Lyu, J. Sun, C. Shang, Y. Cheng, L. Xu. Recent progress on laser absorption spectroscopy for determination of gaseous chemical species. Appl. Spectrosc. Rev.(2020).
[5] R. W. Solarz, J. A. Paisner. Laser Spectroscopy and Its Applications(2017).
[6] Z. Du, S. Zhang, J. Li, N. Gao, K. Tong. Mid-infrared tunable laser-based broadband fingerprint absorption spectroscopy for trace gas sensing: a review. Appl. Sci., 9, 338(2019).
[7] A. Genner, P. Martín-Mateos, H. Moser, B. Lendl. A quantum cascade laser-based multi-gas sensor for ambient air monitoring. Sensors, 20, 1850(2020).
[8] K. Krzempek, G. Dudzik, A. Hudzikowski, A. Gluszek, K. Abramski. Highly-efficient fully-fiberized mid-infrared differential frequency generation source and its application to laser spectroscopy. Opto-Electron. Rev., 25, 269(2017).
[9] N. Picqué, T. W. Hänsch. Frequency comb spectroscopy. Nat. Photon., 13, 146(2019).
[10] F. Adler, K. C. Cossel, M. J. Thorpe, I. Hartl, M. E. Fermann, J. Ye. Phase-stabilized, 1.5 W frequency comb at 2.8–4.8 µm. Opt. Lett., 34, 1330(2009).
[11] T. W. Neely, T. A. Johnson, S. A. Diddams. High-power broadband laser source tunable from 3.0 µm to 4.4 µm based on a femtosecond Yb:fiber oscillator. Opt. Lett., 36, 4020(2011).
[12] T. A. Johnson, S. A. Diddams. Mid-infrared upconversion spectroscopy based on a Yb:fiber femtosecond laser. Appl. Phys. B, 107, 31(2012).
[13] A. Ruehl, A. Gambetta, I. Hartl, M. E. Fermann, K. S. E. Eikema, M. Marangoni. Widely-tunable mid-infrared frequency comb source based on difference frequency generation. Opt. Lett., 37, 2232(2012).
[14] G. Soboń, T. Martynkien, P. Mergo, L. Rutkowski, A. Foltynowicz. High-power frequency comb source tunable from 2.7 to 4.2 µm based on difference frequency generation pumped by an Yb-doped fiber laser. Opt. Lett., 42, 1748(2017).
[15] L. Jin, V. Sonnenschein, M. Yamanaka, H. Tomita, T. Iguchi, A. Sato, K. Nozawa, K. Yoshida, S.-I. Ninomiya, N. Nishizawa. 3.1–5.2 µm coherent MIR frequency comb based on Yb-doped fiber laser. IEEE J. Sel. Top. Quantum Electron., 24, 0900907(2018).
[16] F. Zhu, H. Hundertmark, A. A. Kolomenskii, J. Strohaber, R. Holzwarth, H. A. Schuessler. High-power mid-infrared frequency comb source based on a femtosecond Er:fiber oscillator. Opt. Lett., 38, 2360(2013).
[17] S. A. Meek, A. Poisson, G. Guelachvili, T. W. Hänsch, N. Picqué. Fourier transform spectroscopy around 3 µm with a broad difference frequency comb. Appl. Phys. B, 114, 573(2014).
[18] F. C. Cruz, D. L. Maser, T. Johnson, G. Ycas, A. Klose, F. R. Giorgetta, I. Coddington, S. A. Diddams. Mid-infrared optical frequency combs based on difference frequency generation for molecular spectroscopy. Opt. Express, 23, 26814(2015).
[19] L. Zhou, Y. Liu, H. Lou, Y. Di, G. Xie, Z. Zhu, Z. Deng, D. Luo, C. Gu, H. Chen, W. Li, W. Li. Octave mid-infrared optical frequency comb from Er:fiber-laser-pumped aperiodically poled Mg: LiNbO3. Opt. Lett., 45, 6458(2020).
[20] X. Liu, A. S. Svane, J. Lægsgaard, H. Tu, S. A. Boppart, D. Turchinovich. Progress in Cherenkov femtosecond fiber lasers. J. Phys. D Appl. Phys., 49, 023001(2016).
[21] C. Gaida, M. Gebhardt, T. Heuermann, F. Stutzki, C. Jauregui, J. Antonio-Lopez, A. Schülzgen, R. Amezcua-Correa, A. Tünnermann, I. Pupeza, J. Limpert. Watt-scale super-octave mid-infrared intrapulse difference frequency generation. Light Sci. Appl., 7, 94(2018).
[22] K. Liu, K. Liu, H. Liang, H. Liang, S. Qu, S. Qu, W. Li, W. Li, X. Zou, X. Zou, Y. Zhang, Q. J. Wang. High-energy mid-infrared intrapulse difference-frequency generation with 5.3% conversion efficiency driven at 3 µm. Opt. Express, 27, 37706(2019).
[23] J. Zhang, K. Fritsch, Q. Wang, F. Krausz, K. F. Mak, O. Pronin. Intra-pulse difference-frequency generation of mid-infrared (2.7–20 µm) by random quasi-phase-matching. Opt. Lett., 44, 2986(2019).
[24] D. M. B. Lesko, H. Timmers, S. Xing, A. Kowligy, A. J. Lind, S. A. Diddams. A six-octave optical frequency comb from a scalable few-cycle erbium fibre laser. Nat. Photon., 15, 281(2021).
[25] K. Krzempek, D. Tomaszewska, A. Głuszek, T. Martynkien, P. Mergo, J. Sotor, A. Foltynowicz, G. Soboń. Stabilized all-fiber source for generation of tunable broadband fCEO-free mid-IR frequency comb in the 7–9 µm range. Opt. Express, 27, 37435(2019).
[26] V. S. de Oliveira, A. Ruehl, P. Masłowski, I. Hartl. Intensity noise optimization of a mid-infrared frequency comb difference-frequency generation source. Opt. Lett., 45, 1914(2020).
[27] A. Foltynowicz, T. Ban, P. Masłowski, F. Adler, J. Ye. Quantum-noise-limited optical frequency comb spectroscopy. Phys. Rev. Lett., 107, 233002(2011).
[28] I. E. Gordon, L. S. Rothman, C. Hill, R. V. Kochanov, Y. Tan, P. F. Bernath, M. Birk, V. Boudon, A. Campargue, K. V. Chance, B. J. Drouin, J.-M. Flaud, R. R. Gamache, J. T. Hodges, D. Jacquemart, V. I. Perevalov, A. Perrin, K. P. Shine, M.-A. H. Smith, J. Tennyson, G. C. Toon, H. Tran, V. G. Tyuterev, A. Barbe, A. G. Császár, V. M. Devi, T. Furtenbacher, J. J. Harrison, J.-M. Hartmann, A. Jolly, T. J. Johnson, T. Karman, I. Kleiner, A. A. Kyuberis, J. Loos, O. M. Lyulin, S. T. Massie, S. N. Mikhailenko, N. Moazzen-Ahmadi, H. S. P. Müller, O. V. Naumenko, A. V. Nikitin, O. L. Polyansky, M. Rey, M. Rotger, S. W. Sharpe, K. Sung, E. Starikova, S. A. Tashkun, J. V. Auwera, G. Wagner, J. Wilzewski, P. Wcisło, S. Yu, E. J. Zak. The HITRAN2016 molecular spectroscopic database. J. Quantum Spectrosc. Radiat. Transf., 203, 3(2017).
[29] R. K. Cole, A. S. Makowiecki, N. Hoghooghi, G. B. Rieker. Baseline-free quantitative absorption spectroscopy based on cepstral analysis. Opt. Express, 27, 37920(2019).
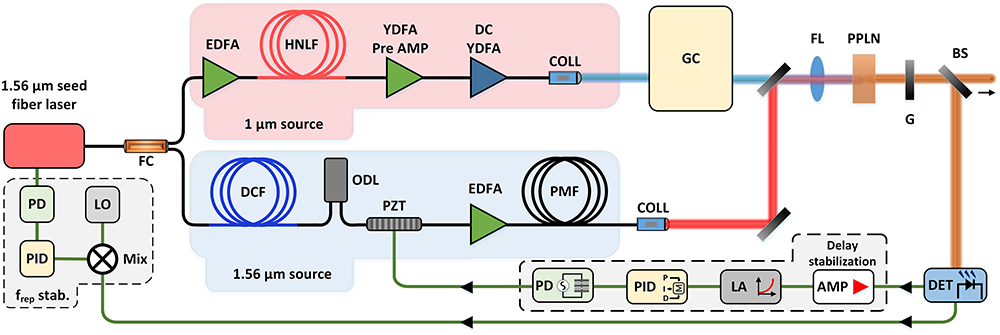
Set citation alerts for the article
Please enter your email address