
- Journal of Semiconductors
- Vol. 42, Issue 4, 041307 (2021)
Abstract
1. Introduction
Driven by the exponential growth of the big data from the internet of things (IoT), 5G, high-performance computing, data center interconnects, and silicon photonics (SiP) has gained tremendous growth as it has the ability to address the high bandwidth and energy efficiency requirements since its first debut back in the 1980s[
Figure 1.(Color online) (a) Evolution of photonic integration in terms of the number of devices in a single PIC. Silicon photonic integration (red circle) represents the “passive” integration without an on-chip laser solution; InP integration (blue squares) and heterogeneous silicon integration (green triangle) are solutions with on-chip lasers[
Many approaches have been proposed in the past few decades, including Raman and Brillouin gain, rare-earth-ion implantation or combining the best of both worlds by heterogeneous integration or direct growth III–V on Si. Among those demonstrations, the most successful and prevalent approach at present is the heterogeneous integration scheme, where III–V material is bonded onto a pre-patterned Si wafer and then being processed together using standard lithography tools as shown in Fig. 1(b). Starting from the University of California, Santa Barbara (UCSB), in 2006[
In this paper, we will review recent progresses on, non-exhaustively, novel light sources and amplifiers on Si based on the two most popular strategies that have been proposed and studied. Section 2 will focus on the narrow linewidth laser-based heterogeneous integration methodology, where a low-loss Si/SiN waveguide provided by the platform can be fully leveraged to improve the optical linewidth. By incorporating the low-loss waveguide as well as high-Q rings inside the cavity, a monolithically integrated semiconductor laser intrinsic linewidth below 200 Hz can be obtained, which is far exceeding the capability of native substrate-based lasers. Section 3 will discuss the potential and benefits provided by the direct epitaxial growth platform, where light sources and optical amplifiers can fully take advantage of the quantum dot (QD) material property. High channel count mode locked lasers (MLLs) and high gain amplifiers will be discussed in more detail. In the final section, we summarize and give a future outlook for this hot research field.
2. Heterogeneously integrated lasers on Si
The strong desire to integrate lasers within large-scale Si photonic integrated circuits (PICs) has pushed the research effort extensively in both academia and industry within the past several decades. Although Si Raman lasers[
Future application scenarios, like coherent communications, on-chip optical sensing, laser gyroscopes, light detection and ranging (LiDAR) systems or precision metrology and timing would require further improvement on the laser performances with an emphasis on relative intensity noise (RIN) and frequency noise (optical linewidth)[
where Γ is the optical confinement factor,
2.1. Ultra-narrow linewidth III–V/Si lasers
Since the laser optical linewidth is fundamentally limited by the ASE noise, the first step to lower the optical linewidth is to decrease the ratio of the ASE noise that is coupled to the oscillating mode inside the laser cavity. By leveraging the heterogeneous silicon laser platform, Santis et al.[
Figure 2.(Color online) High-
A further improvement in both optical linewidth and output power could be obtained by incorporating a low loss Si external cavity. In order to keep the single mode operation, an on-chip filter with ultra-narrow full width half maximum (FWHM) bandwidth is needed. Recently Huang et al.[
Figure 3.(Color online) (a) High-
2.2. Fully integrated III–V/Si/SiN lasers
A recent study has suggested that for high-Q Si resonators (Q > 10 6, radius ~ 100 µm), the nonlinear loss will start to dominate the total loss at even a relatively low power level (a few milliwatts in the bus waveguide)[
The difficulty to realize an efficient III–V/Si3N4 laser lies in the large refractive index difference between those two materials (∆n ~ 1.3−1.5). Even extreme tapering of the thick III–V epitaxial layer is unable to facilitate efficient mode coupling between them within a III–V/Si3N4 structure. Chao et al.[
Figure 4.(Color online) (a) III–V/Si/Si3N4 laser schematic diagram. (b) Si–Si3N4 taper as well as the simulated mode profile. (c) Single-mode optical spectrum with gain current of 160 mA. The inset shows measured normalized reflection spectra of the Si3N4 spiral grating[
2.3. Heterogeneous integrated III–V/Si optical amplifiers
As the silicon PICs continue to scale to larger, more complex configurations, one of the biggest issues is the accumulated insertion loss from those passive switches, waveguide crossings, and couplers, which would put high power requirements on the light source and cause problems with loss due to two photon absorption[
In terms of the SOA design, several figures of merit include the small signal gain factor
Similar to the lasers bonded on Si, the SOAs can be realized with the same epi design and fabrication process[
3. Monolithically integrated QD lasers and amplifiers on Si
Over the past five years, there has been tremendous progress in this area to directly grow III–Vs on Si[
Figure 5.(Color online) (a) Schematic illustration of the typical epitaxial structure used for lasers and amplifiers including one period of the p-modulation doped active region and the III–V/Si buffer including defect filter layers and thermal cycle annealing (TCA) to reduce dislocation densities. (b) As-grown photoluminescence spectra for quantum dot lasers on GaAs and Si substrates[
Previous reports of direct growth methods on Si are mainly based on the 4°–6° offcut Si substrate in order to suppress antiphase domains, which comes from the combination of polar and non-polar bonds. In 2011, University College London (UCL) reported the first electrically pumped 1.3 μm InAs QD laser that was grown directly on a Si (001) substrate with a 4° off-cut[
3.1. Mode locked QD lasers
MLLs can generate wide coherent combs, which consist of equally spaced optical lines in the frequency domain[
Figure 6.(Color online) (a) Schematic diagram of the 20 GHz quantum dot mode-locked laser on silicon (not to scale). (b) Optical spectrum and corresponding optical linewidth of each mode within 10 dB. (c) Relative intensity noise of the whole O-band spectrum and certain filtered individual wavelength channels. (d) BER performance of the PAM-4 signal with different comb lines[
Typical mode locked lasers utilize a two-section structure, where the laser cavity is separated into two sections by an electrical isolation, one gain section for mode amplification and one saturable absorber (SA) section for pulse formation. When in operation, the gain section will be forward current biased and the SA section will be reverse voltage biased. Due to the nonlinear absorption effect of the SA, a pulse will be formed. Recently, several interesting findings reveal that by using a single section gain structure based on the QD material, a pulse can also be formed inside the cavity[
3.2. High gain QD amplifiers
In terms of SOA, QD material has shown multiple advantages compared to its bulk or QW counterparts[
Figure 7.(Color online) Si-based QD-SOA (a) on-chip gain (TE polarization) mapping as a function of on-chip input power and wavelength at 20 °C. (b) On-chip small signal gain as a function of wavelength. (c) On-chip output power as a function of on-chip input power. (d) Wall-plug efficiency as a function of on-chip input power[
4. Conclusions
In this paper, latest progresses on high-performance light sources and amplifiers integrated on silicon based on the heterogeneous or direct growth method have been reviewed. Several detailed examples are given, including narrow linewidth lasers, mode-locked lasers and high gain amplifiers. In general, heterogeneous integration at present offers the greatest flexibility and scalability in terms of the PIC integration capability, where multiple III/V dies can be arbitrarily bonded at any position on a 300 mm silicon wafer. Commercialization is driving its maturity into the product level. Recent results on the hertz-level linewidth semiconductor lasers leveraging CMOS-ready ultra-high-Q microresonators[
Acknowledgements
The authors would like to thank all the members of the UCSB Prof. John Bowers group for the material and useful discussions.
References
[1] A Rahim, T Spuesens, R Baets et al. Open-access silicon photonics: Current status and emerging initiatives. Proc IEEE, 106, 2313(2018).
[2] T Pinguet, S Denton, S Gloeckner et al. High-volume manufacturing platform for silicon photonics. Proc IEEE, 106, 2281(2018).
[3] X Chen, M M Milosevic, S Stanković et al. The emergence of silicon photonics as a flexible technology platform. Proc IEEE, 106, 2101(2018).
[4] M Glick, N C Abrams, Q X Cheng et al. PINE: photonic integrated networked energy efficient datacenters (ENLITENED program). J Opt Commun Netw, 12, 443(2020).
[5]
[6] T Komljenovic, D N Huang, P Pintus et al. Photonic integrated circuits using heterogeneous integration on silicon. Proc IEEE, 106, 2246(2018).
[7]
[8] D Liang, J E Bowers. Recent progress in lasers on silicon. Nat Photonics, 4, 511(2010).
[9] A W Fang, H Park, O Cohen et al. Electrically pumped hybrid AlGaInAs-silicon evanescent laser. Opt Express, 14, 9203(2006).
[10] R Jones, P Doussiere, J B Driscoll et al. Heterogeneously integrated InP\/silicon photonics: Fabricating fully functional transceivers. IEEE Nanotechnol Mag, 13, 17(2019).
[11] A Y Liu, J Bowers. Photonic integration with epitaxial III–V on silicon. IEEE J Sel Top Quantum Electron, 24, 6000412(2018).
[12] J C Norman, D Jung, Y T Wan et al. Perspective: The future of quantum dot photonic integrated circuits. APL Photonics, 3, 030901(2018).
[13] H S Rong, S B Xu, Y H Kuo et al. Low-threshold continuous-wave Raman silicon laser. Nat Photonics, 1, 232(2007).
[14] J F Liu, X C Sun, R Camacho-Aguilera et al. Ge-on-Si laser operating at room temperature. Opt Lett, 35, 679(2010).
[15] Z C Wang, A Abbasi, U Dave et al. Novel light source integration approaches for silicon photonics. Laser Photonics Rev, 11, 1700063(2017).
[16] A W Fang, B R Koch, K G Gan et al. A racetrack mode-locked silicon evanescent laser. Opt Express, 16, 1393(2008).
[17] Z C Wang, K van Gasse, V Moskalenko et al. A III-V-on-Si ultra-dense comb laser. Light: Sci Appl, 6, e16260(2017).
[18] C Zhang, S Srinivasan, Y Tang et al. Low threshold and high speed short cavity distributed feedback hybrid silicon lasers. Opt Express, 22, 10202(2014).
[19] D Liang, X Huang, G Kurczveil et al. Integrated finely tunable microring laser on silicon. Nat Photonics, 10, 719(2016).
[20] T Komljenovic, S Srinivasan, E Norberg et al. Widely tunable narrow-linewidth monolithically integrated external-cavity semiconductor lasers. IEEE J Sel Top Quantum Electron, 21, 214(2015).
[21] G Kurczveil, M J R Heck, J D Peters et al. An integrated hybrid silicon multiwavelength AWG laser. IEEE J Sel Top Quantum Electron, 17, 1521(2011).
[22] C Zhang, S J Zhang, J D Peters et al. 8 × 8 × 40 Gbps fully integrated silicon photonic network on chip. Optica, 3, 785(2016).
[23] G Roelkens, L Liu, D Liang et al. III-V/silicon photonics for on-chip and intra-chip optical interconnects. Laser Photonics Rev, 4, 751(2010).
[24] M A Tran, D N Huang, J E Bowers. Tutorial on narrow linewidth tunable semiconductor lasers using Si/III-V heterogeneous integration. APL Photonics, 4, 111101(2019).
[25] C Henry. Theory of the linewidth of semiconductor lasers. IEEE J Quantum Electron, 18, 259(1982).
[26] M L Davenport, S T Liu, J E Bowers. Integrated heterogeneous silicon/III–V mode-locked lasers. Photon Res, 6, 468(2018).
[27] C T Santis, S T Steger, Y Vilenchik et al. High-coherence semiconductor lasers based on integral high-Q resonators in hybrid Si/III-V platforms. Proc Natl Acad Sci USA, 111, 2879(2014).
[28] M Tran, D N Huang, T Komljenovic et al. Ultra-low-loss silicon waveguides for heterogeneously integrated silicon/III-V photonics. Appl Sci, 8, 1139(2018).
[29] J F Bauters, M J R Heck, D John et al. Ultra-low-loss high-aspect-ratio Si3N4 waveguides. Opt Express, 19, 3163(2011).
[30]
[31] D N Huang, M A Tran, J Guo et al. High-power sub-kHz linewidth lasers fully integrated on silicon. Optica, 6, 745(2019).
[32] B Liu, A Shakouri, J E Bowers. Passive microring-resonator-coupled lasers. Appl Phys Lett, 79, 3561(2001).
[33] A Malik, J Guo, M A Tran et al. Widely tunable, heterogeneously integrated quantum-dot O-band lasers on silicon. Photon Res, 8, 1551(2020).
[34] C Xiang, W Jin, J Guo et al. Effects of nonlinear loss in high-Q Si ring resonators for narrow-linewidth III-V/Si heterogeneously integrated tunable lasers. Opt Express, 28, 19926(2020).
[35]
[36] C Xiang, W Jin, J Guo et al. Narrow-linewidth III-V/Si/Si3N4 laser using multilayer heterogeneous integration. Optica, 7, 20(2020).
[37] C O de Beeck, B Haq, L Elsinger et al. Heterogeneous III-V on silicon nitride amplifiers and lasers via microtransfer printing. Optica, 7, 386(2020).
[38] H Park, C Zhang, M A Tran et al. Heterogeneous silicon nitride photonics. Optica, 7, 336(2020).
[39] Q X Cheng, M Bahadori, M Glick et al. Recent advances in optical technologies for data centers: A review. Optica, 5, 1354(2018).
[40]
[41] T W Berg, J Mork. Saturation and noise properties of quantum-dot optical amplifiers. IEEE J Quantum Electron, 40, 1527(2004).
[42] H Park, A W Fang, O Cohen et al. A hybrid AlGaInAs–silicon evanescent amplifier. IEEE Photonics Technol Lett, 19, 230(2007).
[43] M L Davenport, S Skendžić, N Volet et al. Heterogeneous silicon/III –V semiconductor optical amplifiers. IEEE J Sel Top Quantum Electron, 22, 78(2016).
[44] S Cheung, Y Kawakita, K Shang et al. Highly efficient chip-scale III –V/silicon hybrid optical amplifiers. Opt Express, 23, 22431(2015).
[45] K van Gasse, R J Wang, G Roelkens. 27 dB gain III–V-on-silicon semiconductor optical amplifier with > 17 dBm output power. Opt Express, 27, 293(2019).
[46] S M Chen, W Li, J Wu et al. Electrically pumped continuous-wave III–V quantum dot lasers on silicon. Nat Photonics, 10, 307(2016).
[47] J C Norman, D Jung, Z Y Zhang et al. A review of high-performance quantum dot lasers on silicon. IEEE J Quantum Electron, 55, 2000511(2019).
[48] S J Pan, V Cao, M Y Liao et al. Recent progress in epitaxial growth of III –V quantum-dot lasers on silicon substrate. J Semicond, 40, 101302(2019).
[49] B Shi, Y Han, Q Li et al. 1.55-
[50] W Q Wei, Q Feng, Z H Wang et al. Perspective: optically-pumped III–V quantum dot microcavity lasers via CMOS compatible patterned Si (001) substrates. J Semicond, 40, 53(2019).
[51] D Jung, Z Y Zhang, J Norman et al. Highly reliable low-threshold InAs quantum dot lasers on on-axis (001) Si with 87% injection efficiency. ACS Photonics, 5, 1094(2018).
[52] A Y Liu, S Srinivasan, J Norman et al. Quantum dot lasers for silicon photonics. Photonics Res, 3, B1(2015).
[53] T Wang, H Liu, A Lee et al. 1.3-
[54] A Y Liu, J Peters, X Huang et al. Electrically pumped continuous-wave 13
[55] S M Chen, M Y Liao, M C Tang et al. Electrically pumped continuous-wave 1.3
[56] Y T Wan, J Norman, Q Li et al. 13
[57] W Q Wei, J Y Zhang, J H Wang et al. Phosphorus-free 1.5
[58] Y T Wan, S Zhang, J C Norman et al. Tunable quantum dot lasers grown directly on silicon. Optica, 6, 1394(2019).
[59] S T Liu, J Norman, M Dumont et al. High-performance O-band quantum-dot semiconductor optical amplifiers directly grown on a CMOS compatible silicon substrate. ACS Photonics, 6, 2523(2019).
[60] S T Liu, X R Wu, D Jung et al. High-channel-count 20 GHz passively mode-locked quantum dot laser directly grown on Si with 4.1 Tbit/s transmission capacity. Optica, 6, 128(2019).
[61] Z Y Zhang, Z Y Zhang, J C Norman et al. Integrated dispersion compensated mode-locked quantum dot laser. Photon Res, 8, 1428(2020).
[62] Y Wang, S M Chen, Y Yu et al. Monolithic quantum-dot distributed feedback laser array on silicon. Optica, 5, 528(2018).
[63] Y T Wan, J C Norman, Y Y Tong et al. Quantum dot lasers: 1.3
[64] B L Chen, Y T Wan, Z Y Xie et al. Low dark current high gain InAs quantum dot avalanche photodiodes monolithically grown on Si. ACS Photonics, 7, 528(2020).
[65] W Q Wei, Q Feng, J J Guo et al. InAs/GaAs quantum dot narrow ridge lasers epitaxially grown on SOI substrates for silicon photonic integration. Opt Express, 28, 26555(2020).
[66] Z Y Zhang, D Jung, J C Norman et al. Linewidth enhancement factor in InAs/GaAs quantum dot lasers and its implication in isolator-free and narrow linewidth applications. IEEE J Sel Top Quantum Electron, 25, 1900509(2019).
[67] W W Chow, Z Y Zhang, J C Norman et al. On quantum-dot lasing at gain peak with linewidth enhancement factor
[68] H M Huang, J N Duan, D Jung et al. Analysis of the optical feedback dynamics in InAs/GaAs quantum dot lasers directly grown on silicon. J Opt Soc Am B, 35, 2780(2018).
[69] M G Thompson, A R Rae, M Xia et al. InGaAs quantum-dot mode-locked laser diodes. IEEE J Sel Top Quantum Electron, 15, 661(2009).
[70] S T Liu, H T Wang, M D Sun et al. AWG-based monolithic 4 × 12 GHz multichannel harmonically mode-locked laser. IEEE Photonics Technol Lett, 28, 241(2016).
[71] J N Kemal, P Marin-Palomo, V Panapakkam et al. WDM transmission using quantum-dash mode-locked laser diodes as multi-wavelength source and local oscillator. 2017 Opt Fiber Commun Conf Exhib OFC, 1(2017).
[72] A S P Khope, M Saeidi, R Yu et al. Multi-wavelength selective crossbar switch. Opt Express, 27, 5203(2019).
[73] A S P Khope, S T Liu, Z Y Zhang et al. 2 λ switch. Opt Lett, 45, 5340(2020).
[74] S T Liu, J C Norman, D Jung et al. Monolithic 9 GHz passively mode locked quantum dot lasers directly grown on on-axis (001) Si. Appl Phys Lett, 113, 041108(2018).
[75] S Liu, D Jung, J C Norman et al. 490 fs pulse generation from passively mode-locked single section quantum dot laser directly grown on on-axis GaP/Si. Electron Lett, 54, 432(2018).
[76] S T Liu, X R Wu, J Norman et al. 100 GHz colliding pulse mode locked quantum dot lasers directly grown on Si for WDM application. Conference on Lasers and Electro-Optics, ATu3P-5(2019).
[77] D Auth, S Liu, J Norman et al. Passively mode-locked semiconductor quantum dot on silicon laser with 400 Hz RF line width. Opt Express, 27, 27256(2019).
[78] X R Wu, S T Liu, D Jung et al. Terabit interconnects with a 20-GHz O-band passively mode locked quantum dot laser grown directly on silicon. Optical Fiber Communication Conference (OFC), W2A-3(2019).
[79] Z G Lu, J R Liu, S Raymond et al. 312-fs pulse generation from a passive C-band InAs/InP quantum dot mode-locked laser. Opt Express, 16, 10835(2008).
[80] F Gao, S Luo, H M Ji et al. Single-section mode-locked 1.55-
[81] R Rosales, S G Murdoch, R T Watts et al. High performance mode locking characteristics of single section quantum dash lasers. Opt Express, 20, 8649(2012).
[82] W W Chow, S T Liu, Z Y Zhang et al. Multimode description of self-mode locking in a single-section quantum-dot laser. Opt Express, 28, 5317(2020).
[83] P Bardella, L L Columbo, M Gioannini. Self-generation of optical frequency comb in single section quantum dot Fabry-Perot lasers: A theoretical study. Opt Express, OE, 25, 26234(2017).
[84] T Akiyama, M Sugawara, Y Arakawa. Quantum-dot semiconductor optical amplifiers. Proc IEEE, 95, 1757(2007).
[85] S T Liu, Y Y Tong, J Norman et al. High efficiency, high gain and high saturation output power quantum dot SOAs grown on Si and applications. Optical Fiber Communication Conference (OFC), 1(2020).
[86] J E Bowers, A Gossard, D Jung et al. Quantum dot photonic integrated circuits on silicon. Conference on Lasers and Electro-Optics, 1(2018).
[87] Y Han, Z Yan, W K Ng et al. Bufferless 1.5
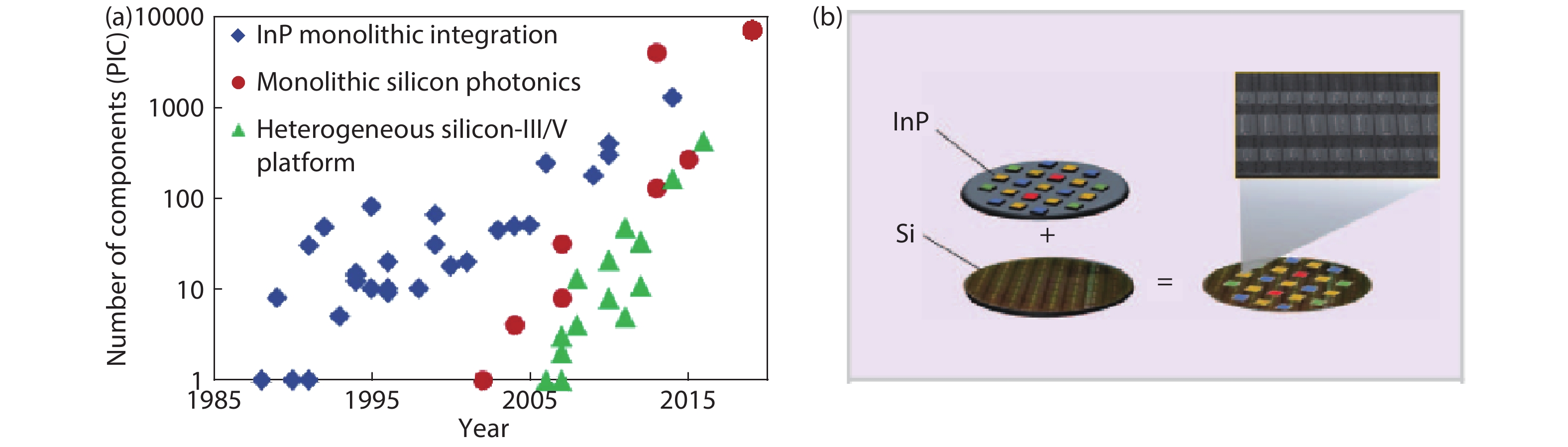
Set citation alerts for the article
Please enter your email address