Abstract
This work studies Te doping effects on the direct bandgap photoluminescence (PL) of indirect alloys (). The temperature-dependent PL shows that the energy difference between direct valley and indirect X valleys is reduced due to the bandgap narrowing (BGN) effect, and the direct band transition gradually dominates the PL spectra as temperature increases. Carrier thermalization has been observed for Te-doped samples, as integrated PL intensity increases with increasing temperature from 175 to 300 K. The activation energy for carrier thermalization is reduced as doping concentration increases. Both BGN effect and carrier thermalization contribute to the carrier injection into the valley. As a result, the direct band transition is enhanced in the Te-doped indirect alloys. Therefore, the PL intensity of the sample with active doping concentration of is increased by five times compared with that of a nominally undoped sample. It is also found that the PL intensity is degraded significantly when the doping concentration is increased to . From cross-section transmission electron microscopy, no large dopant clusters or other extended defects were found contributing to this degradation.1. INTRODUCTION
Large bandgap alloys have potential use for top junction in tandem solar cells and yellow-green light emitting diodes [1,2]. However, the alloy becomes an indirect band alloy when its band energy is above 2.2 eV. At the direct–indirect band crossover, the Ga content of is within a range between 0.69 and 0.72 [3]. Due to the weak luminescence emission, studies of the optical properties of alloys near the direct–indirect band crossover and in the indirect band region are more challenging than for direct band alloys. In addition, the lack of lattice-matched substrates and ordering effects adds more complexity to the studies.
Previous studies have provided detailed information on the electronic structures of alloys () [4,5]. The experimental results showed that the luminescence efficiency of -based LED degrades significantly for emission wavelengths shorter than 590 nm (2.1 eV) [6]. Despite carrier confinement issues for large bandgap alloys [7,8], a simplistic approach was developed to model the luminescence intensity degradation as a function of the energetic separation between direct and indirect bands [9]. Based on this model, as the Ga content x increases for direct band to approach a larger bandgap energy, the energetic separation between the and the X minima, , becomes smaller. Because the density of states (DOS) of the X valley is about 10 times larger than that of the valley of (), the probability of electrons occupying the indirect band minimum is high. Therefore, the luminescence efficiency drops exponentially for alloys near the direct–indirect crossover, and indirect band alloys have poor luminescence efficiency. Although the indirect band alloys have large direct band energies, they are not suitable for light-emitting applications, as indirect band transitions dominate.
More recently, the concept of “pseudo-direct” bandgap has been developed for Ge by using tensile strain and high n-type doping to promote the direct-band transition [10]. The tensile strain reduces the energetic separation between and L valleys in Ge, and carrier injection into the valley was increased. The high n-type doping increased band filling in the L valleys to promote the thermal excitation of electrons into the valley. As a result, strain and doping effects enhance the direct band transition in Ge. The direct band transition dominates the light emission at room temperature, and Ge-based LEDs and lasers have been demonstrated [10,11]. For the indirect alloys near the direct–indirect band crossover, n-type doping is also expected to enhance the direct band transition due to similar mechanisms.
Sign up for Photonics Research TOC. Get the latest issue of Photonics Research delivered right to you!Sign up now
In this paper, we study the n-type Te doping effects on the photoluminescence (PL) of indirect band alloys. The energy difference between and X valleys of the chosen alloys in this study () is less than 40 meV. Temperature-dependent PL provides the basis for a detailed analysis of the doping effects on the electronic structure of the Te-doped samples. This work is meant to explore the indirect alloys for direct band light emission. This work also can be extended for other III–V alloys [, , , etc.] with indirect bandgap or near the direct-indirect band crossover.
2. EXPERIMENTAL
The epitaxy of films on Si (001) substrates off-cut 6° toward the [110] direction was carried out using an Aixtron Crius metal-organic chemical vapor deposition (MOCVD) reactor, where trimethylgallium (TMGa), trimethylindium (TMIn), arsine (), phosphine (), and diethyltellurium (DETe) were used as precursors. A compositionally graded buffer with a grading rate of was used to reach lattice-matching conditions for the films. The -graded buffer was terminated with a 1 μm thick capping layer, which was closely lattice matched (mismatch ) to the films. Then a chemical mechanical polishing (CMP) process was applied to remove the top 500 nm capping layer and planarize the surface. A 100 nm inter-layer was grown on the CMPed capping layer to initiate the III–V growth, which was also lattice matched to the films. Details of the epitaxy of inter-layer on graded buffer on Si (001) can be found in Ref. [12]. A 400 nm film was grown on at 650°C with a V/III ratio of 200. The effective flow rates of TMGa, TMIn, and were 1.31, 0.69, and 400 sccm, respectively, which were the same for all growth runs of films targeting a Ga content of 0.74. The tellurium was in situ doped during the epitaxy of the films, and the effective flow rate of DETe was adjusted for each sample to introduce different Te-doping concentrations. Capacitance-voltage measurements showed that the active doping concentration, n, increased from to , depending on the DETe flow rate. All samples were pieces of the size of .
X-ray diffraction (XRD) was used to measure the strain status and the compositions of the films. Selective defect etching was used to estimate the etch pit density (EPD) of the samples. The selective etching was carried out by pouring concentrated liquid (85 wt. %) into a glass crucible on a hotplate. The hotplate was then heated up and stabilized at the desired temperature of 250°C for 2 min [13]. The samples were lowered into the hot for 10 s. The EPD was counted using a scanning electron microscope (SEM). Temperature-dependent PL measurements were conducted from 6 to 300 K using a 473 nm solid-state laser for excitation. The laser spot size was , and the excitation power was 50 mW. The PL emission was detected using a Spex 750M spectrometer and a Hamamatsu thermoelectrically cooled GaAs photomultiplier tube (PMT).
3. RESULTS AND DISCUSSION
Our target Ga content was 0.74, and XRD measurements confirmed that there is a small tensile strain (0.1%–0.2%) in the undoped films, and the Ga content was measured to be . However, a composition change was observed for the n-type doped sample with , where the Ga content was reduced to 0.720. The XRD results were consistent with secondary ion mass spectrometry measurements. The composition change was caused by the tellurium dopants acting as surfactant, which changed the gallium and indium incorporation into the crystal lattice [14]. In addition, XRD showed that the Ga content was 0.734 for the sample with a doping level of . This suggests that the composition shift was not reproducible, which cannot be simply resolved by increasing the TMGa flow to compensate for the Ga content reduction. In addition, due to the composition shift, strains varied from to 0.25% for Te-doped samples with no clear correlation with Te-doping concentrations.
In Fig. 1(a), the [110]-pole transmission electron microscopy (TEM) pattern for a lightly Te-doped sample () shows only fundamental reflections, even for long exposure times. The absence of superlattice reflections indicates that the sample was fully disordered [15]. The absence of disorder is due to the high growth temperature of 650°C, which is similar to reported in Ref. [16], effectively suppressing the ordering effect in alloys. Furthermore, increased Te-doping concentration also reduces the ordering effect [17]. Therefore, the band energy distortion due to an ordering effect can be eliminated. Figure 1(b) shows the SEM image of the etch pits on the film. The EPD was counted to be , which indicates good epitaxy quality.
![(a) [110]-pole TEM pattern of fully disordered lightly Te-doped Ga0.74In0.26P sample with n=7×1016 cm−3. (b) SEM image of the etch pits of Ga0.74In0.26P film, and EPD=(3±0.5)×106 cm−2.](/richHtml/prj/2017/5/3/03000239/img_001.jpg)
Figure 1.(a) [110]-pole TEM pattern of fully disordered lightly Te-doped sample with . (b) SEM image of the etch pits of film, and .
Temperature-dependent PL is well suited to evaluate materials with direct and indirect bandgap properties. For indirect bandgap semiconductors, the dominant low temperature () PL peak with the highest energy can be attributed to excitonic recombination, while the lower energy peaks are associated with phonon replica and donor-acceptor pair (DAP) recombinations. The excitonic recombination can overlap with band-to-band transitions at higher temperatures, where the band-to-band emission peaks show a redshift with increasing temperature [18]. Direct bandgap semiconductors, however, generally show dominant direct bandgap recombination at low temperatures, as the exciton binding energy is usually quite small [19]. At higher temperatures, the direct bandgap luminescence redshifts and the PL intensity decreases. In the case of an indirect bandgap semiconductor with small difference between direct and indirect bandgap transition, at low temperatures the PL spectrum look like that from an indirect bandgap material while at higher temperatures direct bandgap characteristics dominate [20]. Because the direct bandgap emission is fueled by thermalized electrons, the direct bandgap PL increases with temperature, opposite to the direct bandgap semiconductor PL intensity [21].
According to these rules, the origins of PL emission peaks in indirect samples can be identified. Three Te-doped samples labeled as S01 (), S02 (), and S03 () were selected for detailed analysis of the doping effects on their optical properties. Figure 2 shows the temperature-dependent normalized PL spectra of these three samples. At a low temperature of 6 K, three major peaks are observed for each sample. The peak with the highest energy and the highest PL intensity was assigned to a non-phonon () indirect transition from the X conduction band edge [3]. The measured separation energies between the second peak and the peak for S01, S02, and S03 samples are 29, 22, and 23 meV, respectively. Then, the second peak was likely to be the longitudinal acoustic () peak, with a calculated phonon energy of 29 meV [3]. It should be noted that the experimental separation energies of S02 and S03 are several meV lower than the calculated phonon energy. This could be caused by either composition change or the bandgap narrowing (BGN) effect [22]. As previously mentioned, we suspect a Ga composition change of 2% in Te-doped samples. But this composition change would only cause a small change () in the phonon energy. Therefore, the Te-doping is likely to cause the reduction of the separation energy between and . The broad third peak at low energy was assigned to a DAP emission. In Fig. 2(a), the peak and the peak gradually weaken and finally disappear at 80 K, while another peak at 2.31 eV appears at 100 K. This new peak starts to dominate the PL emission as temperature increases, as indicated by a red arrow. The peak position and its shift to a longer wavelength with increasing temperature indicates that this emission is due to the direct band recombination. The direct band recombination appears at 60 K in S02 mixing with the and peaks, as shown in Fig. 2(b). In Fig. 2(c), the direct band emission peak is inseparable from exciton and photon peaks. The peak broadening from 40 to 60 K indicates the appearance of direct band recombination, and the direct band recombination starts to dominate above 60 K.
![Temperature-dependent normalized PL spectra (6–300 K) of Te-doped Ga0.74In0.26P samples with (a) n=7×1016 cm−3, (b) n=9×1017 cm−3, and (c) n=2×1018 cm−3. The positions of NPx, LAx, and DAP emission peaks from Ref. [3] are labeled, and their positions are indicated by dashed black lines. Solid red lines and arrows indicate the positions of direct band emission, shifting with increasing temperature.](/Images/icon/loading.gif)
Figure 2.Temperature-dependent normalized PL spectra (6–300 K) of Te-doped samples with (a) , (b) , and (c) . The positions of , , and DAP emission peaks from Ref. [3] are labeled, and their positions are indicated by dashed black lines. Solid red lines and arrows indicate the positions of direct band emission, shifting with increasing temperature.
Figure 3 shows the peak positions of the band transitions (squares), lines (circles), and lines (triangles) versus temperature for S01, S02, and S03. The stars present the peak positions deduced from Ref. [3], which show that the data points of the fully disordered lightly doped sample (S01) are closely matched with the reported values. The separation energies between and X bands of S01 and S02 are 39 and 27 meV, respectively. In S02, relative to S01, the BGN effect lowered the peak energies of , , and by , , and , respectively. This shows that the doping-induced BGN effect is more significant for the band shift than for the X band shift. As a result, the separation energy between and X bands is reduced by approximately 10–27 meV. The reduction of the separation energy would lead to an increase of electron injection density in the valley contributing to the enhancement of the PL intensity.
![Peak positions of Te-doped Ga0.74In0.26P samples versus temperature. The data points marked with green stars are from Ref. [3]. Dashed lines are fitted to derive the thermal coefficients of the Γ band.](/Images/icon/loading.gif)
Figure 3.Peak positions of Te-doped samples versus temperature. The data points marked with green stars are from Ref. [3]. Dashed lines are fitted to derive the thermal coefficients of the band.
As previously mentioned for S03, the peak broadening and blueshift from 40 K were due to the direct band emission. In Fig. 3, the temperature coefficients of the band, , are derived from linear regression fitting of the peak energies in the temperature range from 100 to 300 K. The temperature coefficients of S01, S02, and S03 have similar values: , , and , respectively. This indicates that the direct band transition dominates the PL spectra from 100 K upward in S03. Due to the BGN effect and the composition fluctuation, the band edges of and X in S03 are very close (less than 10 meV).
Direct band materials, such as the () alloy, show a thermal quenching process, and its PL intensity decreases as temperature increases [23]. For the indirect alloy, S01, the thermal quenching effect is not obvious, as shown in Fig. 4(a), and the PL spectra are weak. However, Figs. 4(b) and 4(c) show that PL intensities of both S02 and S03 increase with the increasing temperature, which are contrary to the PL in direct and indirect III–V materials. This phenomenon was reported from PL measurements of a 0.22% tensile strained, n-type doped Ge-on-Si. Ge is a well-known indirect band material, and L valley is the minimum of the conduction band. Due to the band-filling effect in the L valleys, the activation energy for direct band transition becomes smaller as doping concentration increases. As a result, more electron carriers are thermally excited from the L valleys to the valley contributing to direct band transition in Ge as temperature increases [21]. The activation energy, , is defined as , where is the direct conduction band edge, and is the Fermi level. The activation energies of S02 and S03 are 79 and 65 meV, respectively, fitted from the Arrhenius plot of the integrated PL intensity versus temperature, as shown in Fig. 5. The direct conduction band edge of S01 is higher than that of S02, as previously mentioned, and its Fermi level is expected lower as it is for lightly -type doping. Thus, the activation energy of S01 is expected to be larger than 110 meV, and carrier thermalization is more difficult to achieve than for in S02. Correlating with Fig. 4, besides high n-type doping concentration, small activation energies of S02 and S03 also enhance the carrier injections into their Γ valleys at room temperature. Additionally, it is also worth mentioning that some publications [24,25] referred the carrier thermalization as a negative thermal quenching effect, and a simplified fitting method was proposed to correlate the PL intensity with the temperature. However, the authors misinterpreted the origins of the observed PL emission peaks; therefore, their approach is not valid.
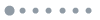
Figure 4.Temperature-dependent PL spectra (175–300 K) of Te-doped samples with (a) S01, , (b) S02, , and (c) S03, .
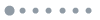
Figure 5.Arrhenius plot of integrated PL intensity versus temperature for Te-doped samples with (red dots) and (blue diamonds). The activation energies, , were derived from the fitted dashed lines.
Figure 6 shows the room-temperature PL intensities and direct band energies of Te-doped samples as a function of active doping concentration. It shows a linear relationship between the band energy reduction and the increase of doping concentration. This observation was also reported for highly n-type doped Ge at room temperature [26]. Although the linear fitting still uses a phenomenological model, it appears to be valid for both n-type doped indirect bandgap Ge and indirect III–V alloys. The direct band energy of the sample with is 10 meV below the fitted curve. According to the numerical results of the major critical points for disordered alloys in Ref. [27], the Ga content reduction of 2% in caused an energy reduction of the , X, and L bands by 11, 4, and 1 meV, respectively. In addition, XRD shows a small compressive strain () in this sample, which is expected to increase its band energy by rather than reducing it. Thus, the composition shift caused band energy reduction agrees well with the discrepancy in the linear fitting of Fig. 6.
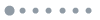
Figure 6.Integrated PL intensity (black squares) of Te-doped samples and their corresponding peak emission energy (blue circles) versus doping concentration at room temperature. Curve fitting (blue solid line) shows an approximate linear regression of emission energy with increasing doping concentration due to BGN effect.
Figure 6 also shows the changes of PL intensities as a function of active doping concentration. The PL spectra were integrated from 2.1 to 2.4 eV. The figure shows that the integrated PL intensity increases with increasing doping concentration. Compared with the lightly doped sample (), the integrated PL intensity of the sample with has increased by approximately five times. Both effects, thermalization and BGN, add to the number of electrons injected into the valley; thus, the PL is enhanced due to the increase in direct band transition. For the sample with , the stronger BGN effect and the composition shift enhances the PL intensity by approximately six times compared with the lightly doped sample.
However, the PL intensity of Te-doped significantly degrades at . This degradation is likely caused by the formation of inactive complexes related to the Te-doping [28]. As tellurium is a large n-type dopant compared with other dopants (i.e., Si), it may distort the sublattices of III–V compounds and form non-radiative recombination centers at high Te-doping concentration. The cross-section TEM (XTEM) image in Fig. 7(a) shows that there are no extended defects found in the film; only some dislocations are visible in the SiGe graded buffer. The high-resolution TEM image in Fig. 7(b) shows no indication of large dopant clusters or other defects. Additionally, TEM inspections under two-beam conditions at and confirmed these observations. Therefore, the Te-doping related defects are likely to be the point defects, which potentially can be detected by deep level transient spectroscopy, as was done for Te-doped GaP [29]. In future work, post-annealing and growth temperature optimization will be explored to suppress the formation of Te-related defects.
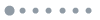
Figure 7.(a) XTEM image of Te-doped sample at . (b) Scanning XTEM image at high resolution.
4. CONCLUSIONS
In conclusion, Te-doped indirect bandgap films were deposited on GaAsP/GeSi/Si. The active doping concentration varied from to . The temperature-dependent PL spectra show that the indirect-to-direct band transition occurs between 40 and 100 K, and the direct band emission dominates the room-temperature PL spectra. Due to the BGN effect, the separation energy between and X bands shrinks as Te-doping concentration increases. Because the activation energy for carrier thermalization is decreased as doping concentration increases, the carrier thermalization is significant in n-type doped indirect bandgap samples with and . Therefore, the doping promotes the carrier injection into the valley, which enhances the direct band transition. We show that the integrated PL intensity has been increased by five times for the sample with compared with the lightly doped sample with . The origin of the PL intensity degradation at high doping concentration is not fully understood, but the TEM results can exclude large dopant clusters. In addition, there is a linear relationship between the BGN and the increasing doping concentration, which agrees with the model used for highly n-type doped Ge.
Acknowledgment
Acknowledgment. This research was supported by the National Research Foundation Singapore through the Singapore MIT Alliance for Research and Technology’s “Low Energy Electronic Systems (LEES) IRG” research. The authors are grateful for the support provided by the management and technical staff at NTU.
References
[1] S. Tomasulo, K. Nay Yaung, J. Faucher, M. Vaisman, M. L. Lee. Metamorphic 2.1–2.2 eV InGaP solar cells on GaP substrates. Appl. Phys. Lett., 104, 173903(2014).
[2] M. J. Mori, S. T. Boles, E. A. Fitzgerald. Comparison of compressive and tensile relaxed composition-graded GaAsP and (Al)InGaP substrates. J. Vac. Sci. Technol. A, 28, 182-188(2010).
[3] K. Alberi, B. Fluegel, M. A. Steiner, R. France, W. Olavarria, A. Mascarenhas. Direct-indirect crossover in GaxIn1−xP alloys. J. Appl. Phys., 110, 113701(2011).
[4] Y. Zhang, C.-S. Jiang, D. J. Friedman, J. F. Geisz, A. Mascarenhas. Tailoring the electronic properties of GaxIn1−xP beyond simply varying alloy composition. Appl. Phys. Lett., 94, 091113(2009).
[5] Y. Ishitani, H. Yaguchi, Y. Shiraki. Temperature dependence of excitonic Γc–Γv transition energies of GaxIn1−xP crystals. Jpn. J. Appl. Phys., 40, 1183-1187(2001).
[6] R. Mueller-Mach, G. O. Mueller, M. R. Krame, O. B. Shchekin, P. J. Schmidt, H. Bechtel, C.-H. Chen, O. Steigelmann. All nitride monochromatic amber emitting phosphor converted light emitting diodes. Phys. Status Solidi, 3, 7-8(2009).
[7] M. J. Mori. Lattice mismatched epitaxy of heterostructures for non-nitride green light emitting devices(2008).
[8] S. J. Chang, C. S. Chang, Y. K. Su, P. T. Chang, Y. R. Wu, K. H. Huang, T. P. Chen. AlGaInP yellow-green light-emitting diodes with a tensile strain barrier cladding layer. IEEE Photon. Technol. Lett., 9, 1199-1201(1997).
[9] G. Oelgart, R. Schwabe, M. Heider, B. Jacobs. Photoluminescence of AlxGa1−xAs near the Γ-X crossover. Semicond. Sci. Technol., 2, 468-474(1987).
[10] J. Liu, X. Sun, D. Pan, X. Wang, L. C. Kimerling, T. L. Koch, J. Michel. Tensile-strained, n-type Ge as a gain medium for monolithic laser integration on Si. Opt. Express, 15, 11272-11277(2007).
[11] X. Sun, J. Liu, L. C. Kimerling, J. Michel. Room-temperature direct bandgap electroluminesence from Ge-on-Si light-emitting diodes. Opt. Lett., 34, 1198-1200(2009).
[12] B. Wang, C. Wang, D. A. Kohen, R. I. Made, K. E. K. Lee, T. Kim, T. Milakovich, E. A. Fitzgerald, S. F. Yoon, J. Michel. Direct MOCVD epitaxy of GaAsP on SiGe virtual substrate without growth of SiGe. J. Cryst. Growth, 441, 78-83(2016).
[13] K. N. Yaung, S. Tomasulo, J. R. Lang, J. Faucher, M. L. Lee. Defect selective etching of GaAsyP1–y photovoltaic materials. J. Cryst. Growth, 404, 140-145(2014).
[14] I. García, I. Rey-Stolle, B. Galiana, C. Algora. Analysis of tellurium as n-type dopant in GaInP: Doping, diffusion, memory effect and surfactant properties. J. Cryst. Growth, 298, 794-799(2007).
[15] M. Steiner, L. Bhusal, J. Geisz. CuPt ordering in high bandgap GaxIn1−xP alloys on relaxed GaAsP step grades. J. Appl. Phys., 106, 063525(2009).
[16] M. J. Mori, E. A. Fitzgerald. Microstructure and luminescent properties of novel InGaP alloys on relaxed GaAsP substrates. J. Appl. Phys., 105, 013107(2009).
[17] S. Jun, G. Stringfellow, A. Howard. Kinetics of Te doping in disodering GaInP grown by organometallic vapor phase epitaxy. J. Appl. Phys., 90, 6048-6053(2001).
[18] M. Guzzi, E. Grilli, S. Oggioni, J. L. Staehli, C. Bosio, L. Pavesi. Indirect-energy-gap dependence on Al concentration in AlxGa1−xAs alloys. Phys. Rev. B, 45, 10951-10957(1992).
[19] Y. P. Varshni. Temperature dependence of the energy gap in semiconductors. Physica, 34, 149-154(1967).
[20] L. Pavesi, M. Guzzi. Photoluminescence of AlxGa1−xAs alloys. J. Appl. Phys., 75, 4779(1994).
[21] X. Sun, J. Liu, L. Kimerling, J. Michel. Direct gap photoluminescence of n-type tensile-strained Ge-on-Si. Appl. Phys. Lett., 95, 011911(2009).
[22] S. C. Jain, D. J. Roulston. A simple expression for band gap narrowing (BGN) in heavily doped Si, Ge, GaAs and GexSi1−x strained layers. Solid State Electron., 34, 453-465(1991).
[23] C. Yang, S. Lee, K. Shin, S. Oh, J. Park. Growth of Si-doped GaInP on Ge-on-Si substrates and its photoluminescence characteristics. Appl. Phys. Lett., 99, 091904(2011).
[24] T. Yokogawa, T. Taguchi, S. Fujita, M. Satoh, M. Satoh. Intense blue-emission band and the fabrication of blue light emitting diodes in I-doped and Ag-ion-implanted cubic ZnS. IEEE Trans. Electron. Devices, 30, 271-277(1983).
[25] H. Shibata. Negative thermal quenching curves in photoluminescence of solids. Jpn. J. Appl. Phys., 37, 550-553(1998).
[26] R. Camacho-Aguilera, Z. Han, Y. Cai, L. C. Kimerling, J. Michel. Direct band gap narrowing in highly doped Ge. Appl. Phys. Lett., 102, 152106(2013).
[27] Y. Zhang, A. Mascarenhas, L. Wang. Interplay of alloying and ordering on the electronic structure of GaxIn1−xP alloys. Phys. Rev. B, 78, 235202(2008).
[28] A. G. Sigai, C. J. Nuese, R. E. Enstrom, T. Zamerowski. Vapor growth of In1−xGaxP for P-N junction electroluminescence. J. Electrochem. Soc., 120, 947-955(1973).
[29] G. E. Zardas, C. I. Symeonides, P. C. Euthymiou, G. J. Papaioannou, P. H. Yannakopoulos, M. Vesely. Electron irradiation induced defects in undoped and Te doped gallium phosphide. Solid State Commun., 145, 332-336(2008).