
- Photonics Research
- Vol. 9, Issue 8, 1439 (2021)
Abstract
1. INTRODUCTION
High-peak-power and average-power lasers with ultrashort pulse width are the enablers for a number of important applications such as wavelength conversions into deep ultraviolet [1], mid-infrared (MIR) [2,3], and terahertz bands [4]; strong-field experiments including high-harmonic generation [5], attosecond pulse generation [6]; and two-dimensional spectroscopy [7]. While titanium-doped sapphire (Ti:sapphire) lasers with high peak power and sub-50 fs pulse width have been routinely employed as the unanimous driving sources, upscaling the average power to the scale
To bridge the gap, a number of Yb gain media such as
One typical application of the sub-50 fs near-infrared driving source with high peak power is the MIR pulse generation via IPDFG. Driving lasers with the pulse width
Sign up for Photonics Research TOC. Get the latest issue of Photonics Research delivered right to you!Sign up now
In this paper, we report a high-power Yb:CALGO regenerative amplification system, delivering 50 fs pulses centered at 1040 nm with 11 W average power and 3.7 GW peak power at a repetition rate of 43 kHz. Combining the ultrabroadband Yb-fiber oscillator, seed spectral shaper, double-end pumping scheme, and Yb:CALGO gain media, 95 fs pulses with 12.5 W average power are output directly from the regenerative amplifier. To the best of our knowledge, this is the first demonstration of sub-100 fs regenerative amplifier based on Yb-doped bulk medium, without nonlinear spectral broadening. In the normal dispersive regime, cascaded-quadratic compression has been a simple and cost effective technique that averts the necessity of expensive chirp mirrors and fine alignment [33,34]. The 95 fs output pulse is further compressed to 50 fs via cascaded-quadratic compression, with 11 W average power and 3.7 GW peak power. Subsequently, MIR emission tuning from 7.5 to 11.5 µm is generated through IPDFG in a long
2. EXPERIMENTAL SETUP
As shown in Fig. 1, the experimental setup consists of four blocks, namely, front end, regenerative amplifier, cascaded-quadratic compressor, and MIR IPDFG stage, with the details presented as follows.
Figure 1.Schematics of (a) front end, (b) regenerative amplifier, (c) cascaded-quadratic compressor, and (d) mid-infrared (MIR) intrapulse difference-frequency generation (IPDFG) stage. The front end consists of an Yb-fiber oscillator and a designed spectral shaper. The regenerative amplifier employs an Yb:CALGO crystal. HR, high reflection mirror; PBS, polarization beam splitter; L, lens; FR, Faraday rotator; HWP, half-wave plate; PC, Pockels cell; QWP, quarter-wave plate; DM, dichroic mirror; LD, laser diode; LPF, low-pass filter; G, high-efficiency transmission grating with 1600 lines/mm; LGS,
A. Front End
In the front end, a customized broadband Yb-fiber oscillator (Yacto-FL-Ultra) with the center wavelength of 1040 nm and
Figure 2.(a) Comparison of the seed spectrum with (red) and without (black) the spectral shaper. The single reflection (blush dash) and overall response (blue dot) of the spectral shaper as a function of the wavelength are also included. (b) Comparison of the amplified spectrum with (red) and without (black) spectral shaping. The spectra correspond to the output power of
B. Regenerative Amplifier
A 1.5%-doped Yb:CALGO crystal with a-cut orientation and 8 mm thickness is mounted on a water-cooled copper holder with four surrounding cooling surfaces. Double-end pumping is adopted by using a fiber-coupled (core diameter of 200 µm) laser diode with a maximum output power of 240 W and the wavelength locked at 980 nm. In the cavity of the regenerative amplifier, which is 2 m in length, telescopes are used to precisely adjust the beam sizes at different optics and to ensure a stable operation. The beam diameters of pump and signal on the Yb:CALGO crystal are designed as
C. Cascaded-Quadratic Compression
To further compress the amplified pulse, an antireflection (AR)-coated BBO crystal with the type I phase-matching angle of 23.5° and 20 mm thickness is used for the cascaded-quadratic compression. The beam size of the output pulse from the Treacy compressor is expanded to 2.6 mm on the BBO with another telescope, generating a peak intensity of
D. MIR IPDFG
With the high-power ultrashort driving pulses, MIR downconversion via IPDFG is also investigated. An AR-coated 8 mm thick LGS crystal with the transparency range covering 0.32–11.6 μm and a bandgap energy of
3. EXPERIMENT RESULTS AND DISCUSSION
A. Sub-100 fs Pulses from Yb Regenerative Amplifier
The seed spectra before and after the spectral shaper are characterized as presented in Fig. 2(a). The seed spectrum from the customized broadband Yb-fiber oscillator spans from 1000 to 1070 nm, which matches well with the emission bandwidth of the Yb:CALGO gain media. With seven bounces from the spectral shaper, the seed spectrum is shaped, forming a spectral hole with
Figure 3.Measured output beam profiles from (a) the Yb:CALGO regenerative amplifier and (b) the cascaded-quadratic compressor.
The amplified pulses from the regenerative amplifier are compressed by a Treacy grating compressor with
Figure 4.SHG-FROG measurement of the 95 fs pulses from the regenerative amplifier. The (a) measured and (b) retrieved FROG traces of the 95 fs pulse. The FROG error is 0.8%. (c) The retrieved temporal profile and the transform-limited temporal profile. (d) The retrieved FROG spectral intensity and phase of the 95 fs laser pulse, compared to the spectrum independently measured using a spectral analyzer.
B. 50 fs Pulses from Cascaded-Quadratic Compression
More advanced applications such as MIR pulse generation via IPDFG require even shorter pulses from high-power regenerative amplifiers. With the cascaded-quadratic compression technique, a long BBO crystal with 20 mm thickness is employed to provide the required phase mismatch in an SHG conversion. The 12.5 W, 290 µJ, 95 fs pulses are incident on the BBO crystal with a peak intensity of
Figure 5.SHG-FROG measurement of the 50 fs pulses from the cascaded-quadratic compressor. The (a) measured and (b) retrieved FROG traces of the 50 fs pulse. The FROG error is 0.95%. (c) The retrieved temporal profile and the transform-limited temporal profile. (d) The retrieved FROG spectral intensity and phase of the 50 fs laser pulse, compared to the spectrum independently measured using a spectral analyzer.
C. MIR Generation in LGS Crystal
For MIR generation via IPDFG, short pulses with a duration of 10–30 fs, compressed by a noble gas filled hollow-core fiber or photonic crystal fiber together with chirp mirrors, have been routinely used as the driving sources. To explore a simpler technique without the necessity of fiber compressors, MIR IPDFG driven by the 50 fs pulses is investigated for the first time to the best of our knowledge. The LGS crystal is chosen for its broad phase-matching bandwidth pumped at
Figure 6.(a) Measured spectra of driving pulses plotted in logarithmic scale from the regenerative amplifier (black), after the BBO cascaded-quadratic compressor (red), and after the 8 mm thick LGS crystal (blue). (b) The measured MIR spectra with different phase-matching angles tuning in the wavelength range of 7.5–11.2 μm.
As measured in Fig. 6(b), by fine-tuning the internal phase-matching angle of the LGS crystal from 51.3° to 49.1°, the MIR emission in the range of 7.5–11.2 μm is generated. The short-wavelength MIR spectrum has broader bandwidth than that of the long-wavelength side, which is attributed to the optimum phase-matching condition centered at
4. CONCLUSION
In conclusion, we demonstrate a high-power Yb:CALGO regenerative amplifier system with 11 W average power, 255 µJ pulse energy, and 50 fs pulse width at 43 kHz repetition rate. The customized Yb-fiber oscillator, specially designed spectral shaper, and Yb:CALGO gain medium with broad emission bandwidth, combined with the cascaded-quadratic compressor, enable ultrashort pulse width and 3.7 GW peak power, which to some extent bridges the gap between the Yb-doped laser and Ti:sapphire laser. It is suggested that there is still large headroom for the power upscaling with the readily available high-power laser diode, large aperture and good thermal conductivity of Yb:CALGO crystal, as well as the self-defocusing nature of the cascaded-quadratic compression. Further amplification of sub-50 fs pulses with
References
[1] K. Liu, H. Li, S. Z. Qu, H. K. Liang, Q. J. Wang, Y. Zhang. 20 W, 2 mJ, sub-ps, 258 nm all-solid-state deep-ultraviolet laser with up to 3 GW peak power. Opt. Express, 28, 18360-18367(2020).
[2] I. Pupeza, D. Sánchez, J. Zhang, N. Lilienfein, M. Seidel, N. Karpowicz, T. Paasch-Colberg, I. Znakovskaya, M. Pescher, W. Schweinberger, V. Pervak, E. Fill, O. Pronin, Z. Wei, F. Krausz, A. Apolonski, J. Biegert. High-power sub-two-cycle mid-infrared pulses at 100 MHz repetition rate. Nat. Photonics, 9, 721-724(2015).
[3] M. Seidel, X. Xiao, S. A. Hussain, G. Arisholm, A. Hartung, K. T. Zawilski, P. G. Schunemann, F. Habel, M. Trubetskov, V. Pervak, O. Pronin, F. Krausz. Multi-watt, multi-octave, mid-infrared femtosecond source. Sci. Adv., 4, eaaq1526(2018).
[4] B. Zhang, Z. Ma, J. Ma, X. Wu, C. Ouyang, D. Kong, T. Hong, X. Wang, P. Yang, L. Chen, Y. Li, J. Zhang. 1.4 mJ high energy terahertz radiation from lithium niobates. Laser Photon. Rev., 15, 2000295(2021).
[5] T. Popmintchev, M. Chen, D. Popmintchev, P. Arpin, S. Brown, S. Ališauskas, G. Andriukaitis, T. Balčiunas, O. D. Mücke, A. Pugzlys, A. Baltuška, B. Shim, S. E. Schrauth, A. Gaeta, C. H. García, L. Plaja, A. Becker, A. J. Becker, M. M. Murnane, H. C. Kapteyn. Bright coherent ultrahigh harmonics in the keV X-ray regime from mid-infrared femtosecond lasers. Science, 336, 1287-1291(2012).
[6] T. Gaumnitz, A. Jain, Y. Pertot, M. Huppert, I. Jordan, F. Ardana-Lamas, H. J. Wörner. Streaking of 43-attosecond soft-X-ray pulses generated by a passively CEP-stable mid-infrared driver. Opt. Express, 25, 27506-27518(2017).
[7] M. Cho. Coherent two-dimensional optical spectroscopy. Chem. Rev., 108, 1331-1418(2008).
[8] V. Cardinali, E. Marmois, B. Le Garrec, G. Bourdet. Determination of the thermo-optic coefficient dn/dT of ytterbium doped ceramics (Sc2O3, Y2O3, Lu2O3, YAG), crystals (YAG, CaF2) and neodymium doped phosphate glass at cryogenic temperature. Opt. Mater., 34, 990-994(2012).
[9] S. Cho, J. Jeong, S. Hwang, T. J. Yu. Thermal lens effect model of Ti:sapphire for use in high-power laser amplifiers related content. Appl. Phys. Express, 11, 092701(2018).
[10] T. Nubbemeyer, M. Kaumanns, M. Ueffing, M. Gorjan, A. Alismail, H. Fattahi, J. Brons, O. Pronin, H. G. Barros, Z. Major, T. Metzger, D. Sutter, F. Krausz. 1 kW, 200 mJ picosecond thin-disk laser system. Opt. Lett., 42, 1381-1384(2017).
[11] Y. Wang, H. Chi, C. Baumgarten, K. Dehne, A. R. Meadows, A. Davenport, G. Murray, B. A. Reagan, C. S. Menoni, J. J. Rocca. 1.1 J Yb:YAG picosecond laser at 1 kHz repetition rate. Opt. Lett., 45, 6615-6618(2020).
[12] B. Schmidt, A. Hage, T. Mans, F. Légaré, H. Wörner. Highly stable, 54 mJ Yb-InnoSlab laser platform at 0.5 kW average power. Opt. Express, 25, 17549-17555(2017).
[13] E. Kaksis, G. Almási, J. A. Fülöp, A. Pugžlys, A. Baltuška, G. Andriukaitis. 110-mJ 225-fs cryogenically cooled Yb:CaF2 multipass amplifier. Opt. Express, 24, 28915-28922(2016).
[14] M. Siebold, S. Bock, U. Schramm, B. Xu, J. L. Doualan, P. Camy, R. Moncorgé. Yb:CaF2 — a new old laser crystal. Appl. Phys. B, 97, 327-338(2009).
[15] G. H. Kim, J. Yang, S. A. Chizov, A. V. Kulik, E. G. Sall, V. E. Yashin, U. Kang. High peak and high average power Yb:KGW laser systems for industrial applications. International Conference Laser Optics, 1(2014).
[16] H. He, J. Yu, W. Zhu, X. Guo, C. Zhou, S. Ruan. A Yb:KGW dual-crystal regenerative amplifier. High Power Laser Sci. Eng., 8, e35(2020).
[17] R. Akbari, A. Major. High-power diode-pumped Kerr-lens mode-locked bulk Yb:KGW laser. Appl. Opt., 56, 8838-8844(2017).
[18] A. Greborio, A. Guandalini, J. Aus der Au. Sub-100 fs pulses with 12.5 W from Yb:CALGO based oscillators. Proc. SPIE, 8235, 823511(2012).
[19] S. Manjooran, A. Major. Diode-pumped 45 fs Yb:CALGO laser oscillator with 1.7 MW of peak power. Opt. Lett., 43, 2324-2327(2018).
[20] E. Caracciolo, A. Guandalini, F. Pirzio, M. Kemnitzer, F. Kienle, A. Agnesi, J. Aus der Au. High power Yb:CALGO ultrafast regenerative amplifier for industrial application. Proc. SPIE, 10082, 100821F(2017).
[21] W. Tian, R. Xu, L. Zheng, X. Tian, D. Zhang, X. Xu, J. Zhu, J. Xu, Z. Wei. 10-W-scale Kerr-lens mode-locked Yb:CALYO laser with sub-100-fs pulses. Opt. Lett., 46, 1297-1300(2021).
[22] A. Rudenkov, V. Kisel, A. Yasukevich, K. Hovhannesyan, A. Petrosyan, N. Kuleshov. Yb:CALYO-based femtosecond chirped pulse regenerative amplifier for temporally resolved pump-probe spectroscopy. Devices Methods Meas., 9, 205-214(2018).
[23] E. Caracciolo, F. Pirzio, M. Kemnitzer, M. Gorjan, A. Guandalini, F. Kienle, A. Agnesi, J. Aus Der Au. 42 W femtosecond Yb:Lu2O3 regenerative amplifier. Opt. Lett., 41, 3395-3398(2016).
[24] I. J. Graumann, A. Diebold, C. G. E. Alfieri, F. Emaury, B. Deppe, M. Golling, D. Bauer, D. Sutter, C. Kränkel, C. J. Saraceno, C. R. Phillips, U. Keller. Peak-power scaling of femtosecond Yb:Lu2O3 thin-disk lasers. Opt. Express, 25, 22519-22536(2017).
[25] P. Loiko, F. Druon, P. Georges, B. Viana, K. Yumashev. Thermo-optic characterization of Yb:CaGdAlO4 laser crystal. Opt. Mater. Express, 4, 2241-2249(2014).
[26] J. Pouysegur, M. Delaigue, Y. Zaouter, C. Hönninger, E. Mottay, A. Jaffrès, P. Loiseau, B. Viana, P. Georges, F. Druon. Sub-100-fs Yb:CALGO nonlinear regenerative amplifier. Opt. Lett., 38, 5180-5183(2013).
[27] B. H. Chen, T. Nagy, P. Baum. Efficient middle-infrared generation in LiGaS2 by simultaneous spectral broadening and difference-frequency generation. Opt. Lett., 43, 1742-1745(2018).
[28] C. Gaida, M. Gebhardt, T. Heuermann, F. Stutzki, C. Jauregui, J. Antonio-Lopez, A. Schülzgen, R. Amezcua-Correa, A. Tünnermann, I. Pupeza, J. Limpert. Watt-scale super-octave mid-infrared intrapulse difference frequency generation. Light Sci. Appl., 7, 94(2018).
[29] J. Zhang, K. F. Mak, N. Nagl, M. Seidel, D. Bauer, D. Sutter, V. Pervak, F. Krausz, O. Pronin. Multi-mW, few-cycle mid-infrared continuum spanning from 500 to 2250 cm−1. Light Sci. Appl., 7, 17180(2018).
[30] S. Vasilyev, I. S. Moskalev, V. O. Smolski, J. M. Peppers, M. Mirov, A. V. Muraviev, P. G. Schunemann, S. B. Mirov, K. L. Vodopyanov, V. P. Gapontsev. Super-octave longwave mid-infrared coherent transients produced by optical rectification of few-cycle 2.5-µm pulses. Optica, 6, 111-114(2019).
[31] Y. G. Jeong, R. Piccoli, D. Ferachou, V. Cardin, M. Chini, S. Hädrich, J. Limpert, R. Morandotti, F. Légaré, B. E. Schmidt, L. Razzari. Direct compression of 170-fs 50-cycle pulses down to 1.5 cycles with 70% transmission. Sci. Rep., 8, 11794(2018).
[32] S. Gröbmeyer, K. Fritsch, B. Schneider, M. Poetzlberger, V. Pervak, J. Brons, O. Pronin. Self-compression at 1 μm wavelength in all-bulk multi-pass geometry. Appl. Phys. B, 126, 159(2020).
[33] F. W. Wise, J. Moses. Self-focusing and self-defocusing of femtosecond pulses with cascaded quadratic nonlinearities. Self-focusing: Past and Present, 114, 481-506(2009).
[34] X. Liu, L. Qian, F. W. Wise. High-energy pulse compression by use of negative phase shifts produced by the cascaded χ(2) : χ(2) nonlinearity. Opt. Lett., 24, 1777-1779(1999).
[35] P. Raybaut, F. Balembois, F. Druon, P. Georges. Numerical and experimental study of gain narrowing in ytterbium-based regenerative amplifiers. IEEE J. Quantum Electron., 41, 415-425(2005).
[36] K. Kato, K. Miyata, L. I. Isaenko, S. Lobanov, V. N. Vedenyapin, V. Petrov. Phase-matching properties of LiGaS2 in the 1.025–10.5910 µm spectral range. Opt. Lett., 42, 4363-4366(2017).
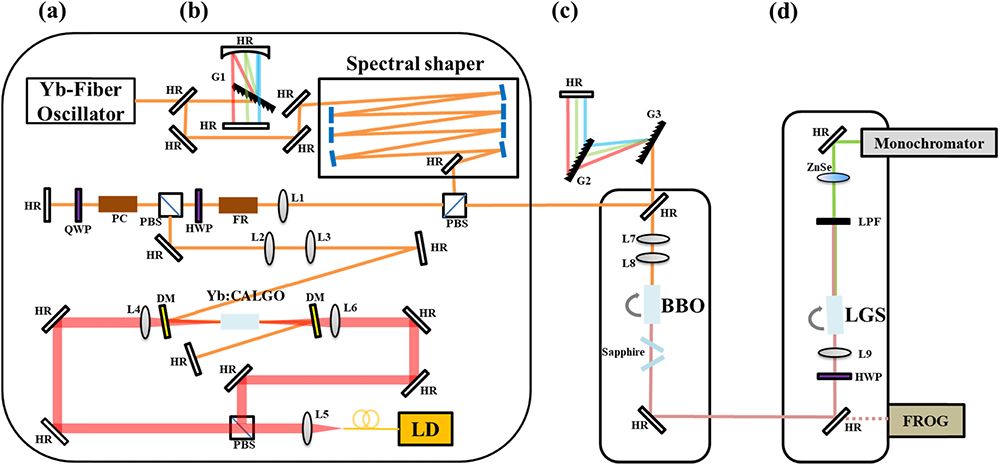
Set citation alerts for the article
Please enter your email address