Fig. 1. Comparison of far- and near-field excitations.
(
a) Far-field (FF) excitation of Si. Here
kphoton,
kc,
kv, and
kphonon are the wave vectors of photon, conduction band, valence band, and phonon, respectively. (
b) Optical near-field (ONF) excitation of Si. Figure is reproduced from ref.
3 under the terms of the Creative Commons Attribution 4.0 International license.
Fig. 2. ONF excitation in indirect band-gap structure.
(
a) Potential, (
b) a schematic of the 1D-KP model, and (
c) dispersion relation. Here,
Eg: band-gap energy and
Ed: direct band-gap energy. Frames (
d) and (
e) show the normalized absorption spectra of the 1D-KP model due to the far- and near-field excitations, respectively. (
f) Absorption spectra due to the electric field components from the dipole radiation, which are
r-3 (red solid line),
r-2 (blue dashed line), and
r-1 (green dotted line). Figure is reprinted with permission from ref.
10, Copyright © 2016 American Physical Society.
Fig. 3. ONF excitation in a realistic Si system.
(
a) The theoretical model consisting of a Si bilayer and the electric dipole measured at a distance of 5 Å above Si surface. (
b) Potential of the ONF induced by the electric dipole shown in (a). (
c) The Fourier transform of (b). (
d) Comparison of the absorption intensity for far-field (blue) and near-field (red) excitations. (
e) Absorption intensity as a function of the variation in the wave vector (∆
k) for the excitation at 1.6 eV. Figure is reproduced from ref.
14 under the terms of the Creative Commons Attribution 4.0 International license.
Fig. 4. Schematic of the hot electron driven photocurrent over a Schottky barrier.
(
a) The Schottky barrier (
ϕB) is lower than the band-gap energy. Therefore, a longer wavelength (
λ2) can be utilized instead of the band-gap wavelength (
λ1). Here, EC: conduction band energy,
EV: valence band energy, and
EF: Fermi level energy. (
b) Three dimensional plasmonic photocapacitor structure. Figure (b) is reprinted with permission from ref.
44, Copyright © 2016 American Chemical Society.
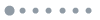
Fig. 5. Sensitivity of the lateral p–n junction with Au nanoparticles.
(
a) Schematic of the device using a Si-on-insulator (SOI). As described in ref.
3, a solution containing Au nanoparticles was dispersed on the device and the solvent was evaporated with a hot plate. We counted this procedure of the dispersion number
N as one. (
b) Scanning electron micrographic (SEM) image of the device. (
c) Magnified SEM image of (b). (
d) Wavelength dependence of sensitivity of the devices. The solid blue circles show the device with the 100-nm Au nanoparticles (
N = 5). The open black circles correspond to the device before the Au nanoparticle dispersion. (
e) Increased photosensitivity rate as a function of the excitation wavelength with different dispersion number
N. Increased rate were obtained as the ratio between the photosensitivity of the device with and without Au nanoparticles. The solid curves show the calculated increased rate using Eq. (7). We used the Au nanoparticle coverage determined by SEM images as the ratios of the direct transition
A:
A=1.82% (
N=10), 0.82% (
N=5), and 0.48% (
N=1). Figure is reproduced from ref.
3 under the terms of the Creative Commons Attribution 4.0 International license.
Fig. 6. Increased rate as a function of the size of Au nanoparticles.
(
a) Increased photosensitivity rate at a wavelength of 1100 nm versus diameter of the Au nanoparticles. (
b) Electric-field intensity distribution
E2 at the interface of Au nanoparticle (diameter
D=100 nm). (
c) Averaged (0 ≤
y≤
D/2) Fourier spectra along x-axis of the electric-field. The wave number difference between the Γ and X points:
kx_ΓX=4.92 nm
-1. (
d) Red solid circles: the diameter dependence of the power spectra at
kx_ΓX= 4.92 (nm
-1), |
F(
E)
ΓX|
2. Blue open circles: the normalized value of the |
F(
E)
ΓX|
2 by the square of the volume, |
F(
E)
ΓX|
2 r-6. Figure is reproduced from ref.
3 under the terms of the Creative Commons Attribution 4.0 International license.