
- Opto-Electronic Advances
- Vol. 4, Issue 7, 200079-1 (2021)
Abstract
Introduction
Supercapacitors, also called electrochemical capacitors or ultracapacitors, have been intensively studied over the past few years to meet the rapidly growing demand for highly efficient energy storage devices
Graphene, a flat monolayer of carbon atoms tightly packed into a two-dimensional (2D) honeycomb lattice, has become a hot research topic since its discovery
In this review, the recent developments of the LSG supercapacitors were summarized. Firstly, we concluded the fabrication and modification of LSG. Particular attention is paid to the application of electrochemical double-layer capacitors (EDLCs), pseudo-capacitors and hybrid supercapacitors, and the diverse strategies to achieve high-performance, flexible, ultrafine-structured and integrated supercapacitors. Current challenges and future advancements of LSG based supercapacitors were also discussed.
Different precursors: graphene oxide, commercial polyimides and other carbon resources were employed for laser scribed graphene production in the literature. The resulting graphene materials are referred differently in scientific reports as laser reduced graphene oxide
Laser scribed graphene
Preparation of laser scribed graphene
Graphene oxide
Graphene oxide (GO), which has the skeleton of graphene decorated with oxygen components, is considered as an important precursor of LSG
Figure 1.
Polymer and biomass
In 2014, Tour’s group reported the fabrication of porous LSG films from commercial polymer films using a CO2 laser
Figure 2.(
Modification of laser scribed graphene
The chemical component, structure and morphology of LSG is strongly affected by the laser scribing process and can be modified by adjusting the laser parameters, laser process, laser system, precursors and environment. Laser systems with varied wavelengths, including CO2 laser (10.6 μm)
Laser scribed graphene based supercapacitor
Based on the different energy storage mechanisms, supercapacitors (SCs) can be divided into two types: electrochemical double-layer capacitors (EDLCs) and pseudo-capacitors
Laser | Precusor | Substrate | Structure | Electrolyte | Supercapacitor performance | Ref. | ||||
Voltage
| CA | CV | EV | PV | ||||||
GO: graphene oxide; PI: polyimide; PET: polyethylene terephthalate; PDMS: polydimethylsiloxane; fs: femtosecond; ns: nanosecond; CNTs: carbon nanotubes; PVA: poly(vinyl alcohol); BMIM-BF4: 1-butyl-3-methylimidazolium tetrafluoroborate; TEABF4: tetraethylammonium tetrafluoroborate; FS-IL: fumed silica nanopowder with the IL 1-butyl-3-methylimidazolium bis(trifluoromethyl sulfonyl)imide; BMIM: 1-butyl-3-methyimidazolium bis (trifluoromethyl sulfonyl) imide; Ni-CAT MOF: Ni-catecholate-based metal–organic frameworks; MnO2: manganese dioxide; FeOOH: ferric oxyhydroxide; PANI: polyaniline | ||||||||||
LightScribe DVD
| GO film | PET | Sandwich | PVA/H3PO4 | 1 | N/A | 0.4 | 0.04 | 1 | Ref. |
TEABF4 | 3 | 4.82 | N/A | 0.4 | 10 | |||||
BMIM-BF4 | 4 | 5.02 | N/A | 0.8 | 10 | |||||
LightScribe DVD
| GO film | PET | In-plane | PVA/H2SO4 | 1 | 2.3 | 3.05 | 0.3 | 60 | Ref. |
FS-IL | 2.5 | N/A | 2.35 | 2 | 150 | |||||
CO2 laser | Hydrated GO film | Free-standing | In-plane | Hydrated GO | 1 | 0.51 | 3.1 | 0.43 | 1.7 | Ref. |
CO2 laser | PI | Free-standing | In-plane | H2SO4 | 1 | 4 | 1.5 | 0.3 | 50 | Ref. |
BMIM-BF4 | 3.5 | 2 | 0.8 | 1 | 100 | |||||
fs 1030 nm laser | GO/HAuCl4 | Paper | In-plane | PVA/H2SO4 | 1 | 0.77 | 17.2 | N/A | N/A | Ref. |
CO2 laser | H3BO3/PI | Free-standing | In-plane | PVA/H2SO4 | 1 | 16.5 | NA | 0.5 | 2 | Ref. |
Laser-scribing DVD burner | CNT/GO | PET | In-plane | PVA/H3PO4 | 1 | N/A | 3.1 | 0.84 | 1 | Ref. |
CO2 laser | PI | Free-standing | In-plane | PVA/H2SO4 | 209 | N/A | 1.43×10-6 | 31.3 | N/A | Ref. |
CO2 laser | PI | Free-standing | Sandwich | PVA/H2SO4 | 1 | 9.11 | N/A | 3 | 2.5 | Ref. |
CO2 laser | GO film | Free-standing | In-plane | BMIM | 2.5 | 270 | N/A | 100 | 100 | Ref. |
CO2 laser | PI-LSG +Ni-CAT MOF | Free-standing | In-plane | PVA/LiCl | 1.4 | 15.2 | N/A | 4.1 | 7 | Ref. |
CO2 laser | PI-LSG +PANI | Free-standing | In-plane | PVA/H2SO4 | 0.8 | 361 | 47.5 | 1.1 | 1.511 | Ref. |
PI-LSG +MnO2 | Free-standing | In-plane | PVA/LiCl | 1 | 934 | 93.4 | 3.2 | 0.298 | ||
PI-LSG +FeOOH/LSG +MnO2 | Free-standing | In-plane asymmetric | PVA/LiCl | 1.8 | 21.9 | 5.4 | 2.4 | 2.891 | ||
CO2 laser | PI-LSG +Fe3O4/
| Free-standing | In-plane asymmetric | PVA/H2SO4 | 1 | 719.28 | 63.04 | 5.3 | 0.02648 | Ref. |
1064 nm laser | PI | Free-standing | In-plane | PVA/LiCl | 1 | 1 | N/A | 1 | 0.02 | Ref. |
Sandwich | 34.77 | 10.21 | 1 | 0.005 | ||||||
355 nm ns laser | GO/Ni | PET | In-plane | PVA/LiCl | 1 | 3.9 | 0.693 | 5.7 | 3 | Ref. |
800 nm fs laser | GO film | Silicon oxide | In-plane | PVA/H2SO4 | 0.5 | 6.3 | 105 | 70 | 10 | Ref. |
800 nm fs laser | GO film | PDMS | In-plane 3D | FS-IL | 2.5 | 0.181 | 0.086 | 100 | 2200 | Ref. |
Table 1. The performances of laser scribed graphene based supercapacitors
LSG based electrochemical double-layer capacitors
In 2012, EI-Kady et al., fabricated the LSG based EDLCs by employing a standard LightScribe DVD optical drive, as shown in Fig. 3(a)
Figure 3.(
Doped LSG for supercapacitors
Doping with heteroatoms (such as boron, nitrogen, phosphorus, and sulfur) has been regarded as an effective way to tailor the electrochemical properties of graphene-derived materials and to enhance their capacitive performances
Figure 4.(
Intercalated LSG for supercapacitors
Owing to the intensive pi–pi interaction of graphene, graphene sheets exhibit the strong tendency to restack together. The restacking issue leads to a significant decrease of ion-accessible surface area and thus a low capacitance of the graphene-based SC
Figure 5.(
Pattern and structure of LSG based supercapacitors
Besides the electrode materials, the structure design of SCs has been also intensively studied to further enhance the performance of devices. Li et al., fabricated a flexible high-voltage LSG-SCs ranging from a few to thousands of volts with a planar in-series architecture, shown in Fig. 6(a)
Figure 6.(
LSG based pseudo-capacitors
Compared to the EDLCs, pseudo-capacitors can achieve much higher capacitances since they store energy through a Faradic process, involving fast and reversible redox reactions between electrolytes and electro-active materials on electrode surfaces
Figure 7.
LSG based hybrid supercapacitor
To maximize the benefits of existing supercapacitors (high power density and stable cycle performance) and lithium-ion batteries (high energy density), hybrid supercapacitors were proposed by Naoi in 2009 by using an asymmetric electrode
Figure 8.(
Featured LSG supercapacitor
Flexible LSG supercapacitor
With the increasing development of wearable devices, flexible energy storage units are highly demanded. Gu et al., reported large-scale flexible LSG supercapacitors with dimension 100 cm2 fabricated on textiles in 3 minutes as shown in Fig. 9(a)
Figure 9.(
Miniaturized LSG supercapacitor
The large dimension of LSG significantly limits the density of graphene electrodes of the supercapacitor, decreases the effective surface area and thus severely deteriorates the energy densities of supercapacitors
Figure 10.(
Integrated LSG supercapacitor
With the recent rapid growth of portable and multifunctional electronic devices, the studies of integrated energy devices have attracted enormous attention. Gu et al. integrated the in-plane supercapacitors with commercial c-Si solar cells by using a CO2 laser to scribe the GO film on the reverse side of solar cells, as shown in Fig. 11(a)
Figure 11.(
Conclusions and outlook
As concluded in this review, great progress has been made in the research field of LSG SCs. LSG can be fabricated from various precursors including GO, polymer and biomass with different laser systems. And its modifications were achieved by adjusting the laser parameters, fabrication processing and environment. The laser direct writing technology can simply induce graphene and simultaneously pattern the graphene electrodes for supercapacitors. Based on LSG, the fabrication of EDLCs, pseudo-capacitors and hybrid supercapacitors and their performances were discussed. Numerous studies have devoted to developing LSG SCs with enhanced performances by element doping, intercalation and pattern optimization. Diverse supercapacitors with advanced features such as being flexible, high power density, miniature, high voltage and new pattern design were also illustrated. The LSG SCs hold great potential for energy storage in the future.
However, there is still room for further improvements. Due to the increasing demand for the portable devices, the miniaturization of energy units is highly expected
Moreover, a broad range of precursors should be explored for LSG, considering not only the properties of LSG but also their impacts on environment and potential for massive production. Various carbon sources, which were traditionally considered as waste, could be reused as precursors of LSG fabrication. The development of potential precursors can effectively utilize resource, reduce pollution and promote the massive production of LSG. In addition, the integration of LSG SCs with energy-generating and energy-consuming components should be further developed. Various energy devices, including thermal or mechanical energy generator, can be investigated as future energy suppliers of LSG SCs. The energy-consuming devices including diverse LSG sensors and LSG transistors can also be integrated. Importantly, these integrated all-LSG devices entirely engraved by laser will facilitate scalable manufacture and the industrialization of LSG SCs. The integration of supercapacitors, solar cells and radiative coolers can also be very attractive. Radiative cooling is a promising cooling method without external energy consumption. The radiative coolers can simultaneously possess a high solar reflection up to 97% and strong infrared emission, cooling the object below ambient temperature
Furthermore, the laser technology should be combined with other advanced technologies. As a significant technique, artificial intelligence (AI) is emerging as an effective approach to solving complicated problems in various fields and is becoming more and more important nowadays
References
[1] XR Zheng, BH Jia, H Lin, L Qiu, D Li et al. Highly efficient and ultra-broadband graphene oxide ultrathin lenses with three-dimensional subwavelength focusing. Nat Commun, 6, 8433(2015).
[2] XW Yun, ZY Xiong, L Tu, LQ Bai, XG Wang. Hierarchical porous graphene film: an ideal material for laser-carving fabrication of flexible micro-supercapacitors with high specific capacitance. Carbon, 125, 308-317(2017).
[3] S Bellani, B Martín-García, R Oropesa-Nuñez, V Romano, L Najafi et al. “Ion sliding” on graphene: a novel concept to boost supercapacitor performance. Nanoscale Horiz, 4, 1077-1091(2019).
[4] MM Wu, YR Li, BW Yao, J Chen, C Li et al. A high-performance current collector-free flexible in-plane micro-supercapacitor based on a highly conductive reduced graphene oxide film. J Mater Chem A, 4, 16213-16218(2016).
[5] CM Chen, Q Zhang, MG Yang, CH Huang, YG Yang et al. Structural evolution during annealing of thermally reduced graphene nanosheets for application in supercapacitors. Carbon, 50, 3572-3584(2012).
[6] GW Huang, N Li, Y Du, QP Feng, HM Xiao et al. Laser-printed in-plane micro-supercapacitors: from symmetric to asymmetric structure. ACS Appl Mater Interfaces, 10, 723-732(2018).
[7] B Zhao, P Liu, Y Jiang, DY Pan, HH Tao et al. Supercapacitor performances of thermally reduced graphene oxide. J Power Sources, 198, 423-427(2012).
[8] YM Chen, MH Guo, L He, W Yang, L Xu et al. Scalable microfabrication of three-dimensional porous interconnected graphene scaffolds with carbon spheres for high-performance all carbon-based micro-supercapacitors. J Mater, 5, 303-312(2019).
[9] AK Geim, KS Novoselov. The rise of graphene. Nat Mater, 6, 183-191(2007).
[10] GJ Li. Direct laser writing of graphene electrodes. J Appl Phys, 127, 010901(2020).
[11] Y Zhao, Q Han, ZH Cheng, L Jiang, LT Qu. Integrated graphene systems by laser irradiation for advanced devices. Nano Today, 12, 14-30(2017).
[12] KS Novoselov, AK Geim, SV Morozov, D Jiang, Y Zhang et al. Electric field effect in atomically thin carbon films. Science, 306, 666-669(2004).
[13] S Bae, H Kim, Y Lee, XF Xu, JS Park et al. Roll-to-roll production of 30-inch graphene films for transparent electrodes. Nat Nanotech, 5, 574-578(2010).
[14] SJ Wang, Y Geng, QB Zheng, JK Kim. Fabrication of highly conducting and transparent graphene films. Carbon, 48, 1815-1823(2010).
[15] ZF Wan, EW Streed, M Lobino, SJ Wang, RT Sang et al. Laser-reduced graphene: synthesis, properties, and applications. Adv Mater Technol, 3, 1700315(2018).
[16] DS Bergsman, BA Getachew, CB Cooper, JC Grossman. Preserving nanoscale features in polymers during laser induced graphene formation using sequential infiltration synthesis. Nat Commun, 11, 3636(2020).
[17] L Guo, HB Jiang, RQ Shao, YL Zhang, SY Xie et al. Two-beam-laser interference mediated reduction, patterning and nanostructuring of graphene oxide for the production of a flexible humidity sensing device. Carbon, 50, 1667-1673(2012).
[18] XP Li, HR Ren, X Chen, J Liu, Q Li et al. Athermally photoreduced graphene oxides for three-dimensional holographic images. Nat Commun, 6, 6984(2015).
[19] MS Loeian, SM Aghaei, F Farhadi, V Rai, HW Yang et al. Liquid biopsy using the nanotube-ctc-chip: capture of invasive ctcs with high purity using preferential adherence in breast cancer patients. Lab Chip, 19, 1899-1915(2019).
[20] W Gao, N Singh, L Song, Z Liu, ALM Reddy et al. Direct laser writing of micro-supercapacitors on hydrated graphite oxide films. Nat Nanotech, 6, 496-500(2011).
[21] T Kavinkumar, P Kavitha, N Naresh, S Manivannan, M Muneeswaran et al. High performance flexible solid-state symmetric supercapacitors based on laser induced porous reduced graphene oxide-graphene oxide hybrid nanostructure devices. Appl Surf Sci, 480, 671-679(2019).
[22] SD Luo, PT Hoang, T Liu. Direct laser writing for creating porous graphitic structures and their use for flexible and highly sensitive sensor and sensor arrays. Carbon, 96, 522-531(2016).
[23] AF Carvalho, AJS Fernandes, C Leitão, J Deuermeier, AC Marques et al. Laser-induced graphene strain sensors produced by ultraviolet irradiation of polyimide. Adv Funct Mater, 28, 1805271(2018).
[24] ZF Wan, M Umer, M Lobino, D Thiel, NT Nguyen et al. Laser induced self-n-doped porous graphene as an electrochemical biosensor for femtomolar miRNA detection. Carbon, 163, 385-394(2020).
[25] ZF Wan, NT Nguyen, YS Gao, Q Li. Laser induced graphene for biosensors. Sustain Mater Technol, 25, e00205(2020).
[26] RZ Li, J Yan, YM Fang, XY Fan, LK Sheng et al. Laser-scribed lossy microstrip lines for radio frequency applications. Appl Sci, 9, 415(2019).
[27] SM Kang, K Lim, H Park, JB Park, SC Park et al. Roll-to-roll laser-printed graphene-graphitic carbon electrodes for high-performance supercapacitors. ACS Appl Mater Interfaces, 10, 1033-1038(2018).
[28] L Zhang, D DeArmond, NT Alvarez, R Malik, N Oslin et al. Flexible micro-supercapacitor based on graphene with 3D structure. Small, 13, 1603114(2017).
[29] JL Ye, HB Tan, SL Wu, K Ni, F Pan et al. Direct laser writing of graphene made from chemical vapor deposition for flexible, integratable micro-supercapacitors with ultrahigh power output. Adva Mater, 30, e1801384(2018).
[30] YN Zhang, L Shi, DJ Hu, SR Chen, SY Xie et al. Full-visible multifunctional aluminium metasurfaces by
[31] H Lin, BH Jia, M Gu. Dynamic generation of debye diffraction-limited multifocal arrays for direct laser printing nanofabrication. Opt Lett, 36, 406-408(2011).
[32] YC Jia, SX Wang, F Chen. Femtosecond laser direct writing of flexibly configured waveguide geometries in optical crystals: fabrica-tion and application. Opto-Electron Adv, 3, 190042(2020).
[33] YL Zhang, L Guo, S Wei, YY He, H Xia et al. Direct imprinting of microcircuits on graphene oxides film by femtosecond laser reduction. Nano Today, 5, 15-20(2010).
[34] ZW Peng, J Lin, RQ Ye, ELG Samuel, JM Tour. Flexible and stackable laser-induced graphene supercapacitors. ACS Appl Mater Interfaces, 7, 3414-3419(2015).
[35] H Wu, WL Zhang, S Kandambeth, O Shekhah, M Eddaoudi et al. Conductive metal–organic frameworks selectively grown on laser‐scribed graphene for electrochemical microsupercapacitors. Adv Energy Mater, 9, 1900482(2019).
[36] R Rahimi, M Ochoa, WY Yu, B Ziaie. Highly stretchable and sensitive unidirectional strain sensor via laser carbonization. ACS Appl Mater Interfaces, 7, 4463-4470(2015).
[37] RK Singh, R Kumar, DP Singh. Graphene oxide: strategies for synthesis, reduction and frontier applications. RSC Adv, 6, 64993-65011(2016).
[38] NQ Deng, H Tian, ZY Ju, HM Zhao, C Li et al. Tunable graphene oxide reduction and graphene patterning at room temperature on arbitrary substrates. Carbon, 109, 173-181(2016).
[39] VA Smirnov, AA Arbuzov, YM Shul’ga, SA Baskakov, VM Martynenko et al. Photoreduction of graphite oxide. High Energy Chem, 45, 57-61(2011).
[40] GC Wang, ZY Yang, XW Li, CZ Li. Synthesis of poly(aniline-co-o-anisidine)-intercalated graphite oxide composite by delamination/reassembling method. Carbon, 43, 2564-2570(2005).
[41] ZF Wan, SJ Wang, B Haylock, J Kaur, P Tanner et al. Tuning the sub-processes in laser reduction of graphene oxide by adjusting the power and scanning speed of laser. Carbon, 141, 83-91(2019).
[42] DW Wang, YG Min, YH Yu, B Peng. Laser induced self-propagating reduction and exfoliation of graphite oxide as an electrode material for supercapacitors. Electrochim Acta, 141, 271-278(2014).
[43] L Huang, Y Liu, LC Ji, YQ Xie, T Wang et al. Pulsed laser assisted reduction of graphene oxide. Carbon, 49, 2431-2436(2011).
[44] Y Hu, HH Cheng, F Zhao, N Chen, L Jiang et al. All-in-one graphene fiber supercapacitor. Nanoscale, 6, 6448-6451(2014).
[45] HH Cheng, MH Ye, F Zhao, CG Hu, Y Zhao et al. A general and extremely simple remote approach toward graphene bulks with in situ multifunctionalization. Adv Mater, 28, 3305-3312(2016).
[46] HH Shi, S Jang, HE Naguib. Freestanding laser-assisted reduced graphene oxide microribbon textile electrode fabricated on a liquid surface for supercapacitors and breath sensors. ACS Appl Mater Interfaces, 11, 27183-27191(2019).
[47] KH Ibrahim, M Irannejad, M Hajialamdari, A Ramadhan, KP Musselman et al. A novel femtosecond laser-assisted method for the synthesis of reduced graphene oxide gels and thin films with tunable properties. Adv Mater Interfaces, 3, 1500864(2016).
[48] J Lin, ZW Peng, YY Liu, F Ruiz-Zepeda, RQ Ye et al. Laser-induced porous graphene films from commercial polymers. Nat Commun, 5, 5714(2014).
[49] ZC Zhang, MM Song, JX Hao, KB Wu, CY Li et al. Visible light laser-induced graphene from phenolic resin: a new approach for directly writing graphene-based electrochemical devices on various substrates. Carbon, 127, 287-296(2018).
[50] LJ Cao, SR Zhu, BB Pan, XY Dai, WW Zhao et al. Stable and durable laser-induced graphene patterns embedded in polymer substrates. Carbon, 163, 85-94(2020).
[51] A Lamberti, M Serrapede, G Ferraro, M Fontana, F Perrucci et al. All-SPEEK flexible supercapacitor exploiting laser-induced graphenization. 2D Mater, 4, 035012(2017).
[52] RQ Ye, Y Chyan, JB Zhang, YL Li, X Han et al. Laser-induced graphene formation on wood. Adv Mater, 29, 1702211(2017).
[53] Y Chyan, RQ Ye, YL Li, SP Singh, CJ Arnusch et al. Laser-induced graphene by multiple lasing: toward electronics on cloth, paper, and food. ACS Nano, 12, 2176-2183(2018).
[54] WL Zhang, YJ Lei, FW Ming, Q Jiang, PMFJ Costa et al. Lignin laser lithography: a direct-write method for fabricating 3D graphene electrodes for microsupercapacitors. Adv Energy Mater, 8, 1801840(2018).
[55] V Strauss, K Marsh, MD Kowal, M El-Kady, RB Kaner. A simple route to porous graphene from carbon nanodots for supercapacitor applications. Adv Mater, 30, 1704449(2018).
[56] JH Zhang, T Zhou, L Wen, AM Zhang. Fabricating metallic circuit patterns on polymer substrates through laser and selective metallization. ACS Appl Mater Interfaces, 8, 33999-34007(2016).
[57] JG Cai, C Lv, A Watanabe. Laser direct writing and selective metallization of metallic circuits for integrated wireless devices. ACS Appl Mater Interfaces, 10, 915-924(2018).
[58] HL Liu, Y Tang, YX Xie, LS Lu, ZP Wan et al. Effect of pulsed ND:YAG laser processing parameters on surface properties of polyimide films. Surf Coat Technol, 361, 102-111(2019).
[59] DF Yang, C Bock. Laser reduced graphene for supercapacitor applications. J Power Sources, 337, 73-81(2017).
[60] A Lamberti, F Perrucci, M Caprioli, M Serrapede, M Fontana et al. New insights on laser-induced graphene electrodes for flexible supercapacitors: tunable morphology and physical properties. Nanotechnology, 28, 174002(2017).
[61] A Tiliakos, C Ceaus, SM Iordache, E Vasile, I Stamatin. Morphic transitions of nanocarbons via laser pyrolysis of polyimide films. J Anal Appl Pyrolysis, 121, 275-286(2016).
[62] TX Tran, H Choi, CH Che, JH Sul, IG Kim et al. Laser-induced reduction of graphene oxide by intensity-modulated line beam for supercapacitor applications. ACS Appl Mater Interfaces, 10, 39777-39784(2018).
[63] XY Fu, YL Zhang, HB Jiang, DD Han, YQ Liu et al. Hierarchically structuring and synchronous photoreduction of graphene oxide films by laser holography for supercapacitors. Opt Lett, 44, 1714-1717(2019).
[64] YC Guan, YW Fang, GC Lim, HY Zheng, MH Hong. Fabrication of laser-reduced graphene oxide in liquid nitrogen environment. Sci Rep, 6, 28913(2016).
[65] YL Li, DX Luong, JB Zhang, YR Tarkunde, C Kittrell et al. Laser-induced graphene in controlled atmospheres: from superhydrophilic to superhydrophobic surfaces. Adv Mater, 29, 1700496(2017).
[66] Y Huang, JJ Liang, YS Chen. An overview of the applications of graphene-based materials in supercapacitors. Small, 8, 1805-1834(2012).
[67] GA Snook, P Kao, AS Best. Conducting-polymer-based supercapacitor devices and electrodes. J Power Sources, 196, 1-12(2011).
[68] LL Zhang, XS Zhao. Carbon-based materials as supercapacitor electrodes. Chem Soc Rev, 38, 2520-2531(2009).
[69] MF El-Kady, V Strong, S Dubin, RB Kaner. Laser scribing of high-performance and flexible graphene-based electrochemical capacitors. Science, 335, 1326-1330(2012).
[70] MF El-Kady, RB Kaner. Scalable fabrication of high-power graphene micro-supercapacitors for flexible and on-chip energy storage. Nat Commun, 4, 1475(2013).
[71] RZ Li, R Peng, KD Kihm, S Bai, D Bridges et al. High-rate in-plane micro-supercapacitors scribed onto photo paper using
[72] ZW Peng, RQ Ye, JA Mann, D Zakhidov, YL Li et al. Flexible boron-doped laser-induced graphene microsupercapacitors. ACS Nano, 9, 5868-5875(2015).
[73] FS Wen, CX Hao, JY Xiang, LM Wang, H Hou et al. Enhanced laser scribed flexible graphene-based micro-supercapacitor performance with reduction of carbon nanotubes diameter. Carbon, 75, 236-243(2014).
[74] XQ Li, WH Cai, KS Teh, MJ Qi, XN Zang et al. High-voltage flexible microsupercapacitors based on laser-induced graphene. ACS Appl Mater Interfaces, 10, 26357-26364(2018).
[75] LV Thekkekara, M Gu. Bioinspired fractal electrodes for solar energy storages. Sci Rep, 7, 45585(2017).
[76] L Li, JB Zhang, ZW Peng, YL Li, CT Gao et al. High-performance pseudocapacitive microsupercapacitors from laser-induced graphene. Adv Mater, 28, 838-845(2016).
[77] HL Liu, KS Moon, JX Li, YX Xie, J Liu et al. Laser-oxidized Fe3O4 nanoparticles anchored on 3D macroporous graphene flexible electrodes for ultrahigh-energy in-plane hybrid micro-supercapacitors. Nano Energy, 77, 105058(2020).
[78] CL Liu, HW Liang, D Wu, XY Lu, Q Wang. Direct semiconductor laser writing of few-layer graphene polyhedra networks for flexible solid-state supercapacitor. Adv Electron Mater, 4, 1800092(2018).
[79] BH Xie, Y Wang, WH Lai, W Lin, ZY Lin et al. Laser-processed graphene based micro-supercapacitors for ultrathin, rollable, compact and designable energy storage components. Nano Energy, 26, 276-285(2016).
[80] DZ Shen, GS Zou, L Liu, WZ Zhao, AP Wu et al. Scalable high-performance ultraminiature graphene micro-supercapacitors by a hybrid technique combining direct writing and controllable microdroplet transfer. ACS Appl Mater Interfaces, 10, 5404-5412(2018).
[81] LV Thekkekara, X Chen, M Gu. Two-photon-induced stretchable graphene supercapacitors. Sci Rep, 8, 11722(2018).
[82] WL Zhang, YJ Lei, Q Jiang, FW Ming, PMFJ Costa et al. 3D laser scribed graphene derived from carbon nanospheres: an ultrahigh‐power electrode for supercapacitors. Small Methods, 3, 1900005(2019).
[83] S Kwon, Y Yoon, J Ahn, H Lim, G Kim et al. Facile laser fabrication of high quality graphene-based microsupercapacitors with large capacitance. Carbon, 137, 136-145(2018).
[84] N Kamboj, T Purkait, M Das, S Sarkar, KS Hazra et al. Ultralong cycle life and outstanding capacitive performance of a 10.8 V metal free micro-supercapacitor with highly conducting and robust laser-irradiated graphene for an integrated storage device. Energy Environ Sci, 12, 2507-2517(2019).
[85] ZW Liu, F Peng, HJ Wang, H Yu, WX Zheng et al. Phosphorus-doped graphite layers with high electrocatalytic activity for the O2 reduction in an alkaline medium. Angew Chem Int Ed, 50, 3257-3261(2011).
[86] Z Yang, Z Yao, GF Li, GY Fang, HG Nie et al. Sulfur-doped graphene as an efficient metal-free cathode catalyst for oxygen reduction. ACS Nano, 6, 205-211(2012).
[87] ZS Wu, A Winter, L Chen, Y Sun, A Turchanin et al. Three-dimensional nitrogen and boron Co-doped graphene for high-performance all-solid-state supercapacitors. Adv Mater, 24, 5130-5135(2012).
[88] XY Fu, DL Chen, Y Liu, HB Jiang, H Xia et al. Laser reduction of nitrogen-rich carbon nanoparticles@graphene oxides composites for high rate performance supercapacitors. ACS Appl Nano Mater, 1, 777-784(2018).
[89] FC Wang, X Dong, KD Wang, WQ Duan, M Gao et al. Laser-induced nitrogen-doped hierarchically porous graphene for advanced electrochemical energy storage. Carbon, 150, 396-407(2019).
[90] HL Liu, YX Xie, JB Liu, KS Moon, LS Lu et al. Laser-induced and KOH-activated 3D graphene: a flexible activated electrode fabricated via direct laser writing for in-plane micro-supercapacitors. Chem Eng J, 393, 124672(2020).
[91] Y Fang, B Luo, YY Jia, XL Li, B Wang et al. Renewing functionalized graphene as electrodes for high‐performance supercapacitors. Adv Mater, 24, 6348-6355(2012).
[92] MH Amiri, N Namdar, A Mashayekhi, F Ghasemi, Z Sanaee et al. Flexible micro supercapacitors based on laser-scribed graphene/ZnO nanocomposite. J Nanopart Res, 18, 237(2016).
[93] SM Lee, YJ Park, JH Kim. Laser reduction of Zn-infiltrated multilayered graphene oxide as electrode materials for supercapacitors. ACS Appl Nano Mater, 2, 3711-3717(2019).
[94] XY Fu, ZD Chen, YL Zhang, DD Han, JN Ma et al. Direct laser writing of flexible planar supercapacitors based on GO and black phosphorus quantum dot nanocomposites. Nanoscale, 11, 9133-9140(2019).
[95] GJ Li, XY Mo, WC Law, KC Chan. 3D printed graphene/nickel electrodes for high areal capacitance electrochemical storage. J Mater Chem A, 7, 4055-4062(2019).
[96] WT Wang, LS Lu, YX Xie, WB Wu, RX Liang et al. Controlling the laser induction and cutting process on polyimide films for kirigami-inspired supercapacitor applications. Sci China Technol Sci, 64, 651-661(2020).
[97] RX Xu, A Zverev, A Hung, CW Shen, L Irie et al. Kirigami-inspired, highly stretchable micro-supercapacitor patches fabricated by laser conversion and cutting. Microsyst Nanoeng, 4, 36(2018).
[98] Y Liang, Z Wang, J Huang, HH Cheng, F Zhao et al. Series of in-fiber graphene supercapacitors for flexible wearable devices. J Mater Chem A, 3, 2547-2551(2015).
[99] Q Liu, QW Shi, HZ Wang, QH Zhang, YG Li. Laser irradiated self-supporting and flexible 3-dimentional graphene-based film electrode with promising electrochemical properties. RSC Adv, 5, 47074-47079(2015).
[100] A Borenstein, V Strauss, MD Kowal, M Yoonessi, M Muni et al. Laser-reduced graphene-oxide/ferrocene: a 3-D redox-active composite for supercapacitor electrodes. J Mater Chem A, 6, 20463-20472(2018).
[101] SH Yang, YY Liu, YF Hao, XP Yang, III Goddard et al. Oxygen-vacancy abundant ultrafine Co3O4/graphene composites for high-rate supercapacitor electrodes. Adv Sci (Weinh), 5, 1700659(2018).
[102] JA Hondred, IL Medintz, JC Claussen. Enhanced electrochemical biosensor and supercapacitor with 3D porous architectured graphene
[103] SH Yang, Y Li, J Sun, BQ Cao. Laser induced oxygen-deficient TiO2/graphene hybrid for high-performance supercapacitor. J Power Sources, 431, 220-225(2019).
[104] A Ladrón-de-Guevara, A Boscá, J Pedrós, E Climent-Pascual, Andrés de et al. Reduced graphene oxide/polyaniline electrochemical supercapacitors fabricated by laser. Appl Surf Sci, 467–468, 691-697(2019).
[105] EC Cho, CW Chang-Jian, WL Syu, HS Tseng, KC Lee et al. PEDOT-modified laser-scribed graphene films as bginder-and metallic current collector-free electrodes for large-sized supercapacitors. Appl Surf Sci, 518, 146193(2020).
[106] K Naoi, S Ishimoto, JI Miyamoto, W Naoi. Second generation ‘nanohybrid supercapacitor’: evolution of capacitive energy storage devices. Energy Environ Sci, 5, 9363-9373(2012).
[107] Pino del, MA Ramadan, PG Lebière, R Ivan, C Logofatu et al. Fabrication of graphene-based electrochemical capacitors through reactive inverse matrix assisted pulsed laser evaporation. Appl Surf Sci, 484, 245-256(2019).
[108] YG Wang, ZD Wang, YY Xia. An asymmetric supercapacitor using RuO2/TiO2 nanotube composite and activated carbon electrodes. Electrochim Acta, 50, 5641-5646(2005).
[109] SH Lee, JH Kim, JR Yoon. Laser scribed graphene cathode for next generation of high performance hybrid supercapacitors. Sci Rep, 8, 8179(2018).
[110] SH Lee, KY Kim, JR Yoon. Binder- and conductive additive-free laser-induced graphene/LiNi1/3Mn1/3Co1/3O2 for advanced hybrid supercapacitors. NPG Asia Mater, 12, 28(2020).
[111] F Clerici, M Fontana, S Bianco, M Serrapede, F Perrucci et al.
[112] LV Thekkekara, M Gu. Large-scale waterproof and stretchable textile-integrated laser- printed graphene energy storages. Sci Rep, 9, 11822(2019).
[113] A Lamberti, F Clerici, M Fontana, L Scaltrito. A highly stretchable supercapacitor using laser-induced graphene electrodes onto elastomeric substrate. Adv Energy Mater, 6, 1600050(2016).
[114] M Parmeggiani, P Zaccagnini, S Stassi, M Fontana, S Bianco et al. PDMS/polyimide composite as an elastomeric substrate for multifunctional laser-induced graphene electrodes. ACS Appl Mater Interfaces, 11, 33221-33230(2019).
[115] CX Shao, T Xu, J Gao, Y Liang, Y Zhao et al. Flexible and integrated supercapacitor with tunable energy storage. Nanoscale, 9, 12324-12329(2017).
[116] J Gao, CX Shao, SX Shao, F Wan, C Gao et al. Laser-assisted large-scale fabrication of all-solid-state asymmetrical micro-supercapacitor array. Small, 14, e1801809(2018).
[117] MG Stanford, C Zhang, JD Fowlkes, A Hoffman, IN Ivanov et al. High-resolution laser-induced graphene. flexible electronics beyond the visible limit. ACS Appl Mater Interfaces, 12, 10902-10907(2020).
[118] R Kumar, R Savu, E Joanni, AR Vaz, MA Canesqui et al. Fabrication of interdigitated micro-supercapacitor devices by direct laser writing onto ultra-thin, flexible and free-standing graphite oxide films. RSC Adv, 6, 84769-84776(2016).
[119] JB In, B Hsia, JH Yoo, S Hyun, C Carraro et al. Facile fabrication of flexible all solid-state micro-supercapacitor by direct laser writing of porous carbon in polyimide. Carbon, 83, 144-151(2015).
[120] ST Wang, YC Yu, RZ Li, GY Feng, ZL Wu et al. High-performance stacked in-plane supercapacitors and supercapacitor array fabricated by femtosecond laser 3D direct writing on polyimide sheets. Electrochim Acta, 241, 153-161(2017).
[121] YJ Yuan, L Jiang, X Li, P Zuo, CY Xu et al. Laser photonic-reduction stamping for graphene-based micro-supercapacitors ultrafast fabrication. Nat Commun, 11, 6185(2020).
[122] LV Thekkekara, BH Jia, YN Zhang, L Qiu, DAN Li et al. On-chip energy storage integrated with solar cells using a laser scribed graphene oxide film. Appl Phys Lett, 107, 031105(2015).
[123] HH Liu, MP Li, RB Kaner, SY Chen, QB Pei. Monolithically integrated self-charging power pack consisting of a silicon nanowire array/conductive polymer hybrid solar cell and a laser-scribed graphene supercapacitor. ACS Appl Mater Interfaces, 10, 15609-15615(2018).
[124] JG Cai, C Lv, A Watanabe. Laser direct writing of high-performance flexible all-solid-state carbon micro-supercapacitors for an on-chip self-powered photodetection system. Nano Energy, 30, 790-800(2016).
[125] SL Kim, JH Hsu, C Yu. Intercalated graphene oxide for flexible and practically large thermoelectric voltage generation and simultaneous energy storage. Nano Energy, 48, 582-589(2018).
[126] Q Liu, ZM Hao, XB Liao, XL Pan, SX Li et al. Langmuir-blodgett nanowire devices for in situ probing of zinc-ion batteries. Small, 15, e1902141(2019).
[127] MM Hossain, M Gu. Radiative cooling: principles, progress, and potentials. Adv Sci (Weinh), 3, 1500360(2016).
[128] MM Hossain, BH Jia, M Gu. A metamaterial emitter for highly efficient radiative cooling. Adv Opt Mater, 3, 1047-1051(2015).
[129] YN Zhang, X Chen, BY Cai, HT Luan, QM Zhang et al. Photonics empowered passive radiative cooling. Adv Photonics Res, 2, 2000106(2020).
[130] W Li, Y Shi, KF Chen, LX Zhu, SH Fan. A comprehensive photonic approach for solar cell cooling. ACS Photonics, 4, 774-782(2017).
[131] M Gu, XY Fang, HR Ren, E Goi. Optically digitalized holography: a perspective for all-optical machine learning. Engineering, 5, 363-365(2019).
[132] E Goi, X Chen, QM Zhang, BP Cumming, S Schoenhardt et al. Nanoprinted high-neuron-density optical linear perceptrons performing near-infrared inference on a CMOS chip. Light: Sci Appl, 10, 40(2021).
[133] E Goi, QM Zhang, X Chen, HT Luan, M Gu. Perspective on photonic memristive neuromorphic computing. PhotoniX, 1, 3(2020).
[134] HR Ren, W Shao, Y Li, F Salim, M Gu. Three-dimensional vectorial holography based on machine learning inverse design. Sci Adv, 6, eaaz4261(2020).
[135] QM Zhang, HY Yu, M Barbiero, BK Wang, M Gu. Artificial neural networks enabled by nanophotonics. Light: Sci Appl, 8, 42(2019).
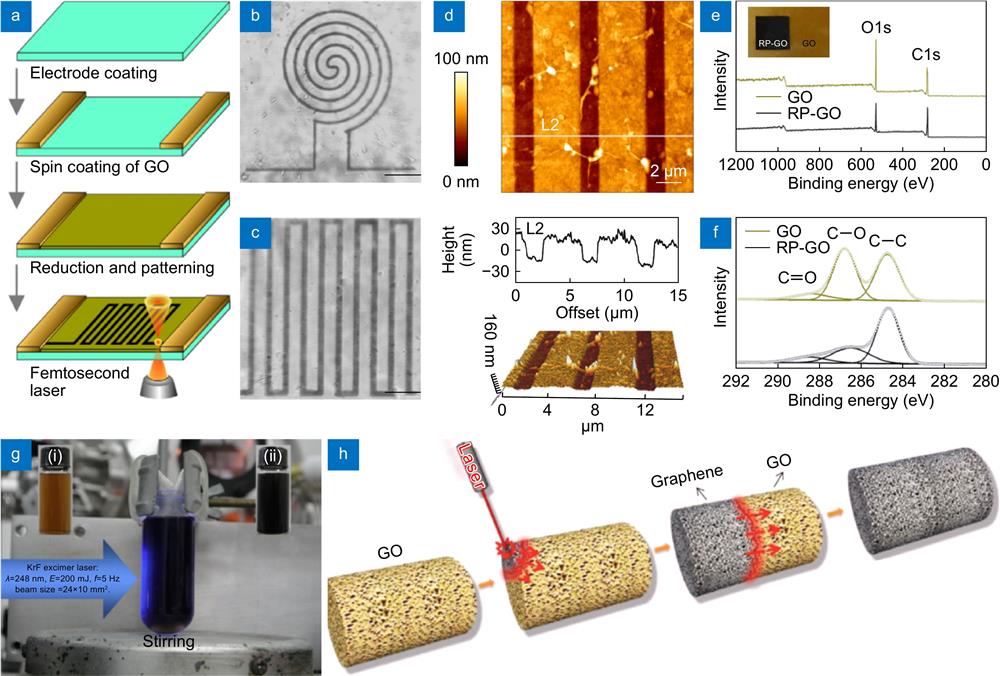
Set citation alerts for the article
Please enter your email address