
- Chinese Optics Letters
- Vol. 20, Issue 2, 021602 (2022)
Abstract
Keywords
1. Introduction
Self-assembled two-dimensional (2D) Ruddlesden–Popper phase organic–inorganic halide perovskites with quantum-well structures have attracted much research attention in optoelectronic devices, lasers, and light-emitting diodes (LEDs) by virtue of their superior features such as enhanced stability, high absorption coefficient, tunable light-emitting wavelength, high color purity, and high exciton binding energy[
In this work, we have fabricated
2. Results and Discussion
To explain the physical mechanism affecting the optical properties of 2D Sn-based perovskites, we prepared
Sign up for Chinese Optics Letters TOC. Get the latest issue of Chinese Optics Letters delivered right to you!Sign up now
Figure 1.Spectral characteristics of (BA)2BI4 (B: Sn/Pb) perovskites. (a) Schematic of the hybrid quantum-well structure of the crystal structure, showing the perovskite octahedra sandwiched between organic spacer molecules (BA+). The UV-visible (UV-Vis) absorption, steady-state photoluminescence (PL) spectra of (b) (BA)2SnI4 and (c) (BA)2PbI4. (d) PLQY of (BA)2SnI4 and (BA)2PbI4.
Figure 2.Analysis of PL characteristics. (a) Multi-peak fit analysis of (BA)2PbI4, (b) multi-peak fit analysis of (BA)2SnI4, (c) percentage of subpeaks of (BA)2PbI4 and (BA)2SnI4, (d) normalized PL decay kinetics.
Figure 3.Temperature dependence of the steady-state PL spectra. Contour map of the temperature-dependent steady-state PL spectra of (a) (BA)2SnI4 and (b) (BA)2PbI4. Fitting of the temperature-dependent FWHM of (c) (BA)2SnI4 and (d) (BA)2PbI4. The red fitting line of the data (blue point) is obtained by Eq. (
The variation of PL FWHM with temperature involves different physical processes of scattering, and the analysis of temperature-dependent PL FWHM is the main means to evaluate the electron-phonon coupling mechanism in various semiconductors[
Sample | ||||
---|---|---|---|---|
Table 1. Best-Fitting Parameters of the (BA)2SnI4 and (BA)2PbI4 Perovskites
Femtosecond TA spectroscopy was utilized to further investigate the photophysical processes of the nonequilibrium interactions of photogenerated carriers[
Figure 4 shows the false-color 2D TA mappings of the
Figure 4.Carrier relaxation dynamics. False-color 2D TA mappings of (a) (BA)2SnI4 and (b) (BA)2PbI4. Evolution-associated spectra (EAS) obtained upon global analysis of the TA data of (c) (BA)2SnI4 and (d) (BA)2PbI4. Decay-associated spectra (DAS) obtained by performing global analysis on the TA spectra of (e) (BA)2SnI4 and (f) (BA)2PbI4. The inset shows the results of fitting the band-edge exciton relaxation dynamics.
The relaxation process of photogenerated carriers at low excitation fluence of
3. Conclusion
In summary, we have fabricated samples
4. Experimental Section
4.1. Syntheses of the (BA)2SnI4 and (BA)2PbI4 perovskites polycrystalline thin films
The glass substrate was cleaned sequentially with detergent, deionized water, ethanol, and isopropanol. Then, the substrate was treated with oxygen plasma for 10 min and dried in an argon flow. For the synthesis of
4.2. Temperature-dependent PL measurement
For temperature-dependent PL measurement, polycrystalline thin films prepared on silica substrates were mounted in a cryostat (Janis ST-100) and cooled by liquid nitrogen. The samples were excited by the continuous wave (CW) laser excitation at a wavelength of 473 nm, power density of 2 µJ/cm2, and 25 K intervals. Fluorescence is separated by the 150 g/mm grating in the Monochromator SP2500 of Princeton Instruments. Then, the spectral information was collected by the PIXIS-100BX CCD at
4.3. Photoluminescence quantum yield
PLQY of polycrystalline thin films prepared on silica substrates was measured using the Edinburgh FLS1000 instrument with an excitation wavelength of 520 nm.
4.4. UV/visible absorption
UV-Vis absorption spectra of polycrystalline thin films prepared on glass substrates were collected by a Lambda 950 UV-Vis spectrometer.
4.5. Time-resolved PL
The TRPL kinetics was detected by a HORIBA DeltaFlex ultrafast time-resolved fluorescence spectrometer, where the excitation wavelength is 405 nm at
References
[1] F. Yuan, X. Zheng, A. Johnston, Y.-K. Wang, C. Zhou, Y. Dong, B. Chen, H. Chen, J. Z. Fan, G. Sharma, P. Li, Y. Gao, O. Voznyy, H.-T. Kung, Z.-H. Lu, O. M. Bakr, E. H. Sargent. Color-pure red light-emitting diodes based on two-dimensional lead-free perovskites. Sci. Adv., 6, eabb0253(2020).
[2] Y. Liu, J. Cui, K. Du, H. Tian, Z. He, Q. Zhou, Z. Yang, Y. Deng, D. Chen, X. Zuo, Y. Ren, L. Wang, H. Zhu, B. Zhao, D. Di, J. Wang, R. H. Friend, Y. Jin. Efficient blue light-emitting diodes based on quantum-confined bromide perovskite nanostructures. Nat. Photonics, 13, 760(2019).
[3] X. Gong, O. Voznyy, A. Jain, W. Liu, R. Sabatini, Z. Piontkowski, G. Walters, G. Bappi, S. Nokhrin, O. Bushuyev, M. Yuan, R. Comin, D. McCamant, S. O. Kelley, E. H. Sargent. Electron-phonon interaction in efficient perovskite blue emitters. Nat. Mater., 17, 550(2018).
[4] A. G. Ricciardulli, S. Yang, J. H. Smet, M. Saliba. Emerging perovskite monolayers. Nat. Mater., 20, 1325(2021).
[5] M. Yuan, L. N. Quan, R. Comin, G. Walters, R. Sabatini, O. Voznyy, S. Hoogland, Y. Zhao, E. M. Beauregard, P. Kanjanaboos, Z. Lu, D. H. Kim, E. H. Sargent. Perovskite energy funnels for efficient light-emitting diodes. Nat. Nanotechnol., 11, 872(2016).
[6] Y. Wang, L. Song, Y. Chen, W. Huang. Emerging new-generation photodetectors based on low-dimensional halide perovskites. ACS Photonics, 7, 10(2019).
[7] Z. L. Cao, F. R. Hu, C. F. Zhang, S. N. Zhu, M. Xiao, X. Y. Wang. Optical studies of semiconductor perovskite nanocrystals for classical optoelectronic applications and quantum information technologies: a review. Adv. Photon., 2, 054001(2020).
[8] J. Wu, R. Su, A. Fieramosca, S. Ghosh, J. Zhao, T. C. H. Liew, Q. Xiong. Perovskite polariton parametric oscillator. Adv. Photon., 3, 055003(2021).
[9] S. Hussain, A. Raza, F. Saeed, A. Perveen, Y. Sikhai, N. Din, E. E. Elemike, Q. Huang, A. Subramanian, Q. Khan, W. Lei. Stable and high performance all-inorganic perovskite light-emitting diodes with anti-solvent treatment. Chin. Opt. Lett., 19, 030005(2021).
[10] L. Jiang, X. Luo, Z. Luo, D. Zhou, B. Liu, J. Huang, J. Zhang, X. Zhang, P. Xu, G. Li. Interface and bulk controlled perovskite nanocrystal growth for high brightness light-emitting diodes [Invited]. Chin. Opt. Lett., 19, 030001(2021).
[11] K. Leng, I. Abdelwahab, I. Verzhbitskiy, M. Telychko, L. Chu, W. Fu, X. Chi, N. Guo, Z. Chen, Z. Chen, C. Zhang, Q. H. Xu, J. Lu, M. Chhowalla, G. Eda, K. P. Loh. Molecularly thin two-dimensional hybrid perovskites with tunable optoelectronic properties due to reversible surface relaxation. Nat. Mater., 17, 908(2018).
[12] D. B. Straus, C. R. Kagan. Electrons, excitons, and phonons in two-dimensional hybrid perovskites: connecting structural, optical, and electronic properties. J. Phys. Chem. Lett., 9, 1434(2018).
[13] J. C. Blancon, J. Even, C. C. Stoumpos, M. G. Kanatzidis, A. D. Mohite. Semiconductor physics of organic-inorganic 2D halide perovskites. Nat. Nanotechnol., 15, 969(2020).
[14] J. C. Blancon, A. V. Stier, H. Tsai, W. Nie, C. C. Stoumpos, B. Traore, L. Pedesseau, M. Kepenekian, F. Katsutani, G. T. Noe, J. Kono, S. Tretiak, S. A. Crooker, C. Katan, M. G. Kanatzidis, J. J. Crochet, J. Even, A. D. Mohite. Scaling law for excitons in 2D perovskite quantum wells. Nat. Commun., 9, 2254(2018).
[15] C. M. Mauck, W. A. Tisdale. Excitons in 2D organic–inorganic halide perovskites. Trends Chem., 1, 380(2019).
[16] T. Palmieri, E. Baldini, A. Steinhoff, A. Akrap, M. Kollar, E. Horvath, L. Forro, F. Jahnke, M. Chergui. Mahan excitons in room-temperature methylammonium lead bromide perovskites. Nat. Commun., 11, 850(2020).
[17] M. Baranowski, P. Plochocka. Excitons in metal-halide perovskites. Adv. Energy Mater., 10, 1903659(2020).
[18] D. H. Cao, C. C. Stoumpos, T. Yokoyama, J. L. Logsdon, T.-B. Song, O. K. Farha, M. R. Wasielewski, J. T. Hupp, M. G. Kanatzidis. Thin films and solar cells based on semiconducting two-dimensional Ruddlesden–Popper (CH3(CH2)3NH3)2(CH3NH3)n-1SnnI3n+1perovskites. ACS Energy Lett., 2, 982(2017).
[19] F. Hao, C. C. Stoumpos, D. H. Cao, R. P. H. Chang, M. G. Kanatzidis. Lead-free solid-state organic–inorganic halide perovskite solar cells. Nat. Photonics, 8, 489(2014).
[20] C. Gao, Y. Jiang, C. Sun, J. Han, T. He, Y. Huang, K. Yao, M. Han, X. Wang, Y. Wang, Y. Gao, Y. Liu, M. Yuan, H. Liang. Multifunctional naphthol sulfonic salt incorporated in lead-free 2D tin halide perovskite for red light-emitting diodes. ACS Photonics, 7, 1915(2020).
[21] J. T. Lin, C. C. Liao, C. S. Hsu, D. G. Chen, H. M. Chen, M. K. Tsai, P. T. Chou, C. W. Chiu. Harnessing dielectric confinement on tin perovskites to achieve emission quantum yield up to 21%. J. Am. Chem. Soc., 141, 10324(2019).
[22] Y. Liao, H. Liu, W. Zhou, D. Yang, Y. Shang, Z. Shi, B. Li, X. Jiang, L. Zhang, L. N. Quan, R. Quintero-Bermudez, B. R. Sutherland, Q. Mi, E. H. Sargent, Z. Ning. Highly oriented low-dimensional tin halide perovskites with enhanced stability and photovoltaic performance. J. Am. Chem. Soc., 139, 6693(2017).
[23] A. Swarnkar, V. K. Ravi, A. Nag. Beyond colloidal cesium lead halide perovskite nanocrystals: analogous metal halides and doping. ACS Energy Lett., 2, 1089(2017).
[24] Y. J. Heo, H. J. Jang, J.-H. Lee, S. B. Jo, S. Kim, D. H. Ho, S. J. Kwon, K. Kim, I. Jeon, J.-M. Myoung, J. Y. Lee, J.-W. Lee, J. H. Cho. Enhancing performance and stability of tin halide perovskite light emitting diodes via coordination engineering of Lewis acid-base adducts. Adv. Funct. Mater., 2106974(2021).
[25] Z. Wang, F. Wang, B. Zhao, S. Qu, T. Hayat, A. Alsaedi, L. Sui, K. Yuan, J. Zhang, Z. Wei, Z. Tan. Efficient two-dimensional tin halide perovskite light-emitting diodes via a spacer cation substitution strategy. J. Phys. Chem. Lett., 11, 1120(2020).
[26] Z. He, Y. Liu, Z. Yang, J. Li, J. Cui, D. Chen, Z. Fang, H. He, Z. Ye, H. Zhu, N. Wang, J. Wang, Y. Jin. High-efficiency red light-emitting diodes based on multiple quantum wells of phenylbutylammonium-cesium lead iodide perovskites. ACS Photonics, 6, 587(2019).
[27] M. D. Smith, B. A. Connor, H. I. Karunadasa. Tuning the luminescence of layered halide perovskites. Chem. Rev., 119, 3104(2019).
[28] M. C. Weidman, M. Seitz, S. D. Stranks, W. A. Tisdale. Highly tunable colloidal perovskite nanoplatelets through variable cation, metal, and halide composition. ACS Nano, 10, 7830(2016).
[29] J. Lee, E. S. Koteles, M. O. Vassell. Luminescence linewidths of excitons in GaAs quantum wells below 150 K. Phys. Rev. B, 33, 5512(1986).
[30] S. Rudin, T. L. Reinecke, B. Segall. Temperature-dependent exciton linewidths in semiconductors. Phys. Rev. B, 42, 11218(1990).
[31] X. B. Zhang, T. Taliercio, S. Kolliakos, P. Lefebvre. Influence of electron-phonon interaction on the optical properties of III nitride semiconductors. J. Phys. Condens. Mat., 13, 7053(2001).
[32] M. D. Smith, H. I. Karunadasa. White-light emission from layered halide perovskites. Acc. Chem. Res., 51, 619(2018).
[33] S. Kahmann, E. K. Tekelenburg, H. Duim, M. E. Kamminga, M. A. Loi. Extrinsic nature of the broad photoluminescence in lead iodide-based Ruddlesden–Popper perovskites. Nat. Commun., 11, 2344(2020).
[34] E. P. Booker, T. H. Thomas, C. Quarti, M. R. Stanton, C. D. Dashwood, A. J. Gillett, J. M. Richter, A. J. Pearson, N. Davis, H. Sirringhaus, M. B. Price, N. C. Greenham, D. Beljonne, S. E. Dutton, F. Deschler. Formation of long-lived color centers for broadband visible light emission in low-dimensional layered perovskites. J. Am. Chem. Soc., 139, 18632(2017).
[35] B. Traore, L. Pedesseau, L. Assam, X. Che, J. C. Blancon, H. Tsai, W. Nie, C. C. Stoumpos, M. G. Kanatzidis, S. Tretiak, A. D. Mohite, J. Even, M. Kepenekian, C. Katan. Composite nature of layered hybrid perovskites: assessment on quantum and dielectric confinements and band alignment. ACS Nano, 12, 3321(2018).
[36] A. Hermann, J. Furthmüller, H. W. Gäggeler, P. Schwerdtfeger. Spin-orbit effects in structural and electronic properties for the solid state of the group-14 elements from carbon to superheavy element 114. Phys. Rev. B, 82, 155116(2010).
[37] R. Saran, A. Heuer-Jungemann, A. G. Kanaras, R. J. Curry. Giant band gap renormalization and exciton phonon scattering in perovskite nanocrystals. Adv. Opt. Mater., 5, 1700231(2016).
[38] A. D. Wright, C. Verdi, R. L. Milot, G. E. Eperon, M. A. Perez-Osorio, H. J. Snaith, F. Giustino, M. B. Johnston, L. M. Herz. Electron-phonon coupling in hybrid lead halide perovskites. Nat. Commun., 7, 11755(2016).
[39] L. Ni, U. Huynh, A. Cheminal, T. H. Thomas, R. Shivanna, T. F. Hinrichsen, S. Ahmad, A. Sadhanala, A. Rao. Real-time observation of exciton-phonon coupling dynamics in self-assembled hybrid perovskite quantum wells. ACS Nano, 11, 10834(2017).
[40] J. Li, J. Hu, J. Ma, X. Wen, D. Li. Identifying self-trapped excitons in 2D perovskites by Raman spectroscopy [Invited]. Chin. Opt. Lett., 19, 103001(2021).
[41] N. Mondal, A. De, S. Das, S. Paul, A. Samanta. Ultrafast carrier dynamics of metal halide perovskite nanocrystals and perovskite-composites. Nanoscale, 11, 9796(2019).
[42] Z. Guo, Y. Wan, M. J. Yang, J. Snaider, K. Zhu, L. B. Huang. Long-range hot-carrier transport in hybrid perovskites visualized by ultrafast microscopy. Science, 356, 59(2017).
[43] J. Chen, C. Zhang, X. Liu, L. Peng, J. Lin, X. Chen. Review on the study of carrier dynamic process in all-inorganic halide perovskites by photoluminescence measurement. Photon. Res., 9, 151(2020).
[44] L. M. Herz. Charge-carrier dynamics in organic-inorganic metal halide perovskites. Annu. Rev. Phys. Chem., 67, 65(2016).
[45] T. Zhang, J. Wang. Defect-enhanced exciton–exciton annihilation in monolayer transition metal dichalcogenides at high exciton densities. ACS Photonics, 8, 2770(2021).
[46] J. S. Manser, P. V. Kamat. Band filling with free charge carriers in organometal halide perovskites. Nat. Photonics, 8, 737(2014).
[47] X. Wu, M. T. Trinh, X. Y. Zhu. Excitonic many-body interactions in two-dimensional lead iodide perovskite quantum wells. J. Phys. Chem. C, 119, 14714(2015).
[48] X. Wu, M. T. Trinh, D. Niesner, H. Zhu, Z. Norman, J. S. Owen, O. Yaffe, B. J. Kudisch, X. Y. Zhu. Trap states in lead iodide perovskites. J. Am. Chem. Soc., 137, 2089(2015).
[49] Z. Guo, Y. Wan, M. Yang, J. Snaider, K. Zhu, L. Huang. Long-range hot-carrier transport in hybrid perovskites visualized by ultrafast microscopy. Science, 356, 59(2017).
[50] X. L. Li, X. Lian, J. H. Pang, B. B. Luo, Y. H. Xiao, M. D. Li, X. C. Huang, J. Z. Zhang. Defect-related broadband emission in two-dimensional lead bromide perovskite microsheets. J. Phys. Chem. Lett., 11, 8157(2020).
[51] H. Shi, R. Yan, S. Bertolazzi, J. Brivio, B. Gao, A. Kis, D. Jena, H. G. Xing, L. Huang. Exciton dynamics in suspended monolayer and few-layer MoS2 2D crystals. ACS Nano, 7, 1072(2013).
[52] Y. Jiang, M. Cui, S. Li, C. Sun, Y. Huang, J. Wei, L. Zhang, M. Lv, C. Qin, Y. Liu, M. Yuan. Reducing the impact of Auger recombination in quasi-2D perovskite light-emitting diodes. Nat. Commun., 12, 336(2021).
[53] Z. Guo, X. Wu, T. Zhu, X. Zhu, L. Huang. Electron–phonon scattering in atomically thin 2D perovskites. ACS Nano, 10, 9992(2016).
[54] J. S. Yao, J. C. Zhang, L. Wang, K. H. Wang, X. C. Ru, J. N. Yang, J. J. Wang, X. Chen, Y. H. Song, Y. C. Yin, Y. F. Lan, Q. Zhang, H. B. Yao. Suppressing Auger recombination in cesium lead bromide perovskite nanocrystal film for bright light-emitting diodes. J. Phys. Chem. Lett., 11, 9371(2020).
[55] M. J. Trimpl, A. D. Wright, K. Schutt, L. R. V. Buizza, Z. Wang, M. B. Johnston, H. J. Snaith, P. Müller-Buschbaum, L. M. Herz. Charge-carrier trapping and radiative recombination in metal halide perovskite semiconductors. Adv. Funct. Mater., 30, 2004312(2020).
[56] K. Miyata, T. L. Atallah, X. Y. Zhu. Lead halide perovskites: crystal-liquid duality, phonon glass electron crystals, and large polaron formation. Sci. Adv., 3, e1701469(2017).
[57] D. Ghosh, A. J. Neukirch, S. Tretiak. Optoelectronic properties of two-dimensional bromide perovskites: influences of spacer cations. J. Phys. Chem. Lett., 11, 2955(2020).
[58] A. Mahata, D. Meggiolaro, L. Gregori, F. De Angelis. Suppression of tin oxidation by 3D/2D perovskite interfacing. J. Phys. Chem. C, 125, 10901(2021).
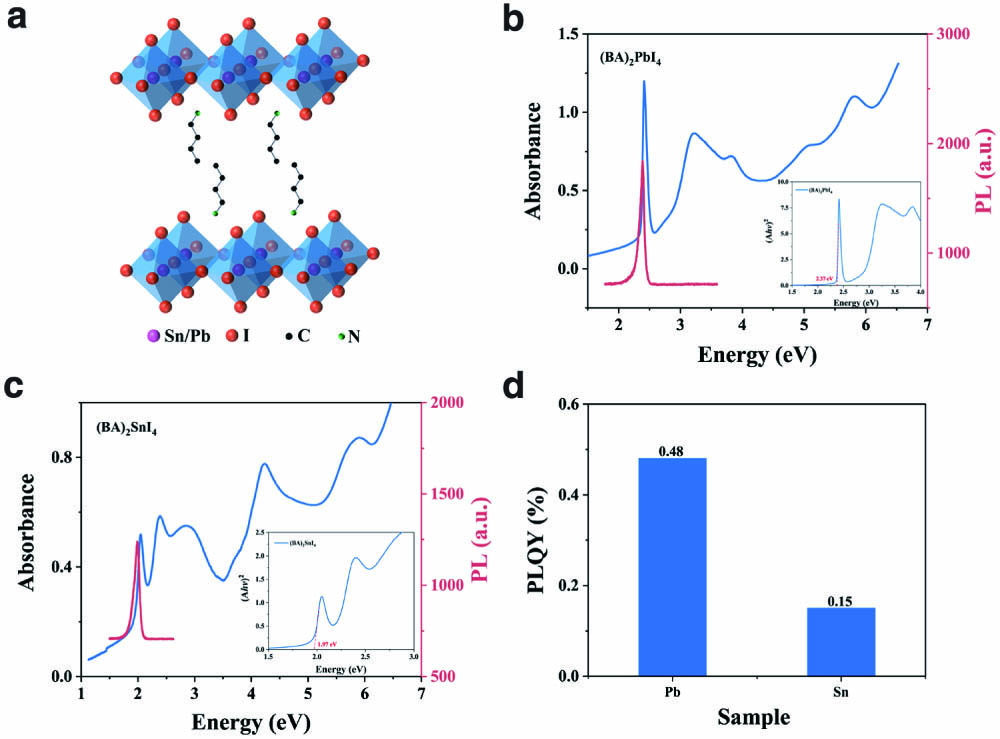
Set citation alerts for the article
Please enter your email address