Fig. 1. Approaches for inducing molecular UDR. (a) Double-pulse scheme for excitation of unidirectional molecular rotation (adapted from Ref.
53, CC BY). (b) A train of linearly polarized laser pulses are spaced in time by a fixed delay
, and the polarization axis rotates between each pulse.
79 (c) Polarization shaping setup. (d), (e) Illustrations of the electric field of the pulse for phase
and
(
), producing a linear “twisted” polarization in the clockwise and counterclockwise directions, respectively. (f) The same for intermediate phase
, resulting in an elliptical polarization in the region of overlap of the two pulses. Panels (c)–(f) are adapted with permission from Ref.
58, © 2020, American Physical Society (APS).
Fig. 2. Illustration of an optical centrifuge pulse. The vector
k denotes the propagation direction of the pulse. The vector of linear polarization
E undergoes an accelerated rotation about the propagation direction. (b) Frequency spectra of a full (dashed) and truncated (solid) centrifuge pulse. (c) Time-frequency spectrogram of the centrifuge field, recorded by means of cross-correlation frequency resolved optical gating. This figure is reproduced with permission from Ref.
63, © 2020, APS.
Fig. 3. Experimentally observed RDS for deuterium molecules. (a)–(c) The spectra, shown as 2-D color-coded plots, were measured with a probe scan delay of 150 fs around the midway point between the pump pulses. (d)–(f) Normalized spectra measured at the probe delay time that produced the maximum signal [indicated by the horizontal gray lines in (a)–(c)]. Vertical black line marks the central wavelength of the unperturbed probe. (a), (d) A single pump pulse is applied, resulting in no UDR and no RDS. (b), (e) The molecules are set to rotate in the same sense as the CP probe, producing a red shift. (c), (f) The molecules are set to rotate in the opposite sense, producing a blue shift. This figure is reproduced with permission from Ref.
80.
Fig. 4. Time-dependent Raman shifts. From the (a) clockwise and (b) counterclockwise centrifuged oxygen molecules. As the molecules spend more time in the centrifuge, the observed Raman frequency shift increases, providing direct evidence of accelerated molecular rotation in one, well defined, direction. An additional time-independent Raman signal originates from the molecules lost from the centrifuge. This figure is reproduced with permission from Ref.
63, © 2020, APS.
Fig. 5. COLTRIMS experimental setup for molecular UDR measurements. The measurement is performed in an ultra-high-vacuum apparatus of COLTRIMS. A supersonic gas jet of nitrogen molecules is impulsively aligned by a linearly polarized (along
axis) pulse, which is subsequently kicked to rotate unidirectionally by a properly matched second pulse (polarized at
in the
plane). An intense circularly polarized probe pulse with adjustable time delay is used to Coulomb explode the molecule allowing the imaging of the evolution of the rotational wave packet. The insets (a)–(f) are the snapshots of the measured momentum distribution of the fragments of the Coulomb exploded nitrogen molecule, and the insets (A)–(F) are the simulated distributions of the rotational wave packet at various time delays. Figure adapted with permission from Ref.
81, © 2020, APS.
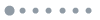
Fig. 6. Time-dependent angular distributions of UDR molecules. (a) Experimentally measured angular distribution of (
,
) fragments in the
plane (see
Fig. 5) ejected from a dissociatively doubly ionized nitrogen molecule as a function of the time delay of the probe pulse. White dashed lines denote the crossed and parallel structures of the angular distribution (see the text). The panel was reproduced with permission from Ref.
81, © 2020, APS. (b) Observed time- and angular-dependent probability, in the rotational wave packet dynamics. Before
, molecules are randomly distributed (image from Ref.
82, CC BY-NC 4.0). In (a) and (b), molecules are randomly distributed before
. (c) Probability density as a function of the molecular angle and the free propagation time of
prepared in the equal-weight superposition of
and
states. The observed nondispersing behavior illustrates the main property of a quantum cogwheel state. The classical period is indicated with the white horizontal bar at the lower right corner. Reproduced from Ref.
105 with permission from the PCCP Owner Societies.
![Field-free enantioselective orientation of PPO molecule. (a) Schematic illustration of the experimental geometry. Cold PPO molecules in a seeded helium jet are spun in an optical centrifuge and Coulomb exploded with a probe pulse between the plates of a conventional VMI spectrometer, equipped with an MCP detector and a phosphor screen. The inset shows the fixed frame axes and the definition of angles θ and θ2-D used in text. (b) (R)-PPO molecule. Atoms are color-coded: black, carbon; gray, hydrogen; and red, oxygen. Principal axes of inertia tensor (I frame) are shown as solid arrows and labeled by a, b, and c (moments of inertia are ordered Ia<Ib<Ic), whereas those of polarizability tensor (A frame) are shown as dashed arrows and labeled by x1, x2, and x3 (principal polarizabilities are ordered α33<α22<α11). Coulomb explosion trajectories from a stationary molecule are shown with thin black and red lines. (c), (d) Experimentally measured degree of 2-D alignment [panel (c)] and orientation [panel (d)] in the velocity distribution of O+. Blue(red) colored markers correspond to the left- (right-)handed enantiomer. The black dashed curves in upper panels show the intensity profile of the centrifuge field in arbitrary units. (e) Binet ellipsoid. Shown in blue are the allowed trajectories of the angular momentum vector. As an example, two trajectories (Ta− and Tc+) are labeled according to the notation used in the text. (f) Experimentally measured 2-D orientation factor in the velocity distribution of O+ fragments as a function of optical centrifuge-probe pulse delay, t. Orange: right handed molecule, (R)-PPO; blue: left handed molecule, (S)-PPO. Note the reversal of colors between the two plots. Panels (a), (b), and (f) are reproduced with permission from Ref. 122. Panels (c) and (d) are reproduced with permission from Ref. 68, © 2020, APS.](/Images/icon/loading.gif)
Fig. 7. Field-free enantioselective orientation of PPO molecule. (a) Schematic illustration of the experimental geometry. Cold PPO molecules in a seeded helium jet are spun in an optical centrifuge and Coulomb exploded with a probe pulse between the plates of a conventional VMI spectrometer, equipped with an MCP detector and a phosphor screen. The inset shows the fixed frame axes and the definition of angles
and
used in text. (b) (R)-PPO molecule. Atoms are color-coded: black, carbon; gray, hydrogen; and red, oxygen. Principal axes of inertia tensor (
frame) are shown as solid arrows and labeled by
,
, and
(moments of inertia are ordered
), whereas those of polarizability tensor (
frame) are shown as dashed arrows and labeled by
,
, and
(principal polarizabilities are ordered
). Coulomb explosion trajectories from a stationary molecule are shown with thin black and red lines. (c), (d) Experimentally measured degree of 2-D alignment [panel (c)] and orientation [panel (d)] in the velocity distribution of
. Blue(red) colored markers correspond to the left- (right-)handed enantiomer. The black dashed curves in upper panels show the intensity profile of the centrifuge field in arbitrary units. (e) Binet ellipsoid. Shown in blue are the allowed trajectories of the angular momentum vector. As an example, two trajectories (
and
) are labeled according to the notation used in the text. (f) Experimentally measured 2-D orientation factor in the velocity distribution of
fragments as a function of optical centrifuge-probe pulse delay,
. Orange: right handed molecule, (R)-PPO; blue: left handed molecule, (S)-PPO. Note the reversal of colors between the two plots. Panels (a), (b), and (f) are reproduced with permission from Ref.
122. Panels (c) and (d) are reproduced with permission from Ref.
68, © 2020, APS.
Fig. 8. Trajectories of the polarization vector tip of the OTC pulse in case (a) when the amplitude of the FW is greater than that of the SH and (b) vice versa. (c) Optical layout for constructing a collinearly propagating two-color field by nonlinear optical-mixing technique. MPA, most polarizable axis and SMPA, second most polarizable axis. Notice that in case of
SMPA is the axis that must be oriented, whereas in case of iodobenzene MPA is the one requiring orientation. Panel (c) is adapted with permission from Ref.
152, © 2020, APS.
Fig. 9. 1-D model describing the OTC pulse-induced orientation. (a)
molecule, the atoms are color coded: sulfur, yellow and oxygen, red. The major axis is perfectly aligned along the laboratory
axis. The minor axis lies in the
plane at an angle
relative to the
axis. (b) Potential energy as a function of
for
, [see Eqs. (3) and (4)]. This figure is from Ref.
146, CC BY 4.0.
Fig. 10. Coincidentally measured momentum distributions of
(vertical panels) and
(horizontal panels). Here
and
are the projections of fragments’ momenta on
and
axes, respectively (measured in atomic units). (a) Isotropic momentum distribution for
and
ions measured before the arrival of OTC pulse. (b), (c) Anisotropic momentum distributions for
and
ions measured at
after the application of the OTC pulse at
and
, respectively. (d), (e) Angular distributions of
derived from (b) and (c), respectively. Gray bars represent the uncertainty propagated during data analysis according to Gaussian’ s propagation law. This figure is from Ref.
146, CC BY 4.0.
Fig. 11. A sequence of two short-orienting pulses
is applied. The pulses are delayed by the time
. After an additional delay that is after
, an impulsive response emerges, denoted
. Higher order echoes appear after
This figure is adapted with permission from Ref.
36, © 2020, APS.
Fig. 12. Filamentation of the phase-space density distribution. In this figure,
. (a) Initial smooth phase space distribution (b) shortly after the kick,
, the density distribution folds, resulting in a transient alignment along the direction
. The folded pattern is centrally symmetric with respect to the phase-space point
that is not affected by the kick. (c) On the longer time scale,
, the probability density becomes wrinkled and develops multiple parallel filaments. (d) After a second kick is applied at
(with
and
), every filament in (c) folds in a manner similar to (b), giving rise to an alignment echo near
at time
after the second kick (
). (e) At
(
), a fractional echo is formed.
refers to time counted from the first pulse, whereas
to time counted from the second pulse. For details, see Ref.
37.
Fig. 13. Left: Hahn’s famous spin echo analogy on the cover of
Physics Today (Ref.
167). Pictorial rotational density matrix representations of (a) an effective two level system invoked by the Raman selection rule (
). (b) Two coherent pathways starting from a mutual population term
and interfere to create the rephasing coherence term
. (c) Two coherent pathways starting from two neighboring population terms
and
that interfere at the rephasing coherence term
. This figure is reproduced with permission from Ref.
168, © 2020, APS.
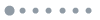
Fig. 14. Left: (a) Birefringence signals as a function of the pump–probe delay
for intensities
and
of the pump pulses
and
, respectively, (see the inset).
, rotational revival time. (b) Third-harmonic signal detected by selecting the harmonic field component parallel to the alignment axis
. The delay
between
and
is set to 2.5 ps. Middle: time-dependent angular distributions of full,
and
echoes, as indicated between the white dashed lines in (c) 2.2 to 3.5 ps, (f) 1.9 to 3.0 ps, and (i) 1.4 to 1.9 ps, experimentally measured and theoretically simulated for
. (c) Carpet of the angular distribution of full echo for parallel input pulses,
and
, with
time delay between them. (d), (e) Polar plots of the alignment and antialignment regions of the distribution for the full echo, showing vertical and horizontal directionality, respectively. (f)–(h) The same, for the
fractional echo, with
time delay. (i)–(k) The same for
fractional echoes with
time delay. In all cases, the solid red line is the quantum-mechanically simulated distribution. Panels (a) and (b) are reproduced with permission from Refs.
36 and
38, respectively. Panels (c)–(k) are from Ref.
37 (CC BY 3.0).
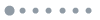
Fig. 15. Experimentally measured time-dependent angular distributions of the rotated echo in
.
and
arrive at
and 0 ps, respectively, with a relative angle
. (a) Angular distribution of the rotated echo as a function of the probe time delay (see the region of 2.2 to 3.5 ps between the white dashed lines). (b) Polar plot of the rotated echo at 3 ps. (c), (d) Experimentally measured time-dependent angular distributions of the imaginary echo in
.
and
arrive at
and 0 ps, respectively. (c) A “carpet” describing the angular distribution of the imaginary echo as a function of the probe time delay (see the region of 36 to 38 ps between the white dashed lines). (d) The alignment factor
as a function of the probe delay (red shows the revival of the response to the first pulse, blue that of the second pulse, green depicts the revival of the first full echo, and purple presents the imaginary echo). This figure is from Ref.
37, CC BY 3.0.
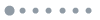
Fig. 16. (a) Experimental results of the maximal echo amplitude (
, marked by blue circles), obtained at various delays between pulses, and the corresponding optimal
intensities (
, green dots) required to induce them. Theoretical simulations of
and of
are depicted by the blue triangles (connected by blue dashed curve) and by the green curve, respectively. Note that the
axis depicts the time of the second pulse (
), and thus the echo signals (with their maximal amplitudes marked by the blue circles) are observed at
. (b) Low-pressure temporal traces of the echo recorded in
for different time delays
between the pulse
and
as a function of the probe delay
defined with respect to
. The intensity of
is set to the optimal value for the delay
, i.e.,
. (c) High-pressure temporal traces in
gas mixture at a pressure of 43.5 bar with an enlargement of the echo decay shown in the inset. The estimated intensity of
is
. The positions of the alignment peaks respective to
and
are marked with arrows. (d) Peak-to-dip amplitude of the echo (filled circles) recorded in a
gas mixture (
bar) as a function of
compared with classical molecular dynamics simulations (CMDS) (dashed line). Least squares fitting of the experimental data with an exponential law (solid line). (e) Decay rates
of the echo (filled circles) measured for various densities of the gas mixture with the linear fit (solid line) of the data and the CMDS calculations (dashed line). The error bars reflect the dispersion of the measurements. The bottom (top)
axis denotes the gas density (pressure) in units of amagat (bar). Panel (a) is reproduced with permission from Ref.
168. Panels (c)–(f) are reproduced with permission from Ref.
174, © 2020, APS.
Fig. 17. Time constants of collisional relaxation of
. The circles with error bars (representing two standard deviations of the mean) are the density-normalized decay time constants
of the echoes deduced from the measurements of the alignment signal recorded at various
gas densities and fixed delays
between the two pulses. The dashed lines denote the results of simulations conducted by solving the density matrix equations for molecules impulsively aligned by two short laser pulses and interacting with each other through collisions. The red dashed lines have been obtained using the standard Bloch equations (i.e., using the secular approximation) and the green dashed line represents the results obtained using the nonsecular Redfield equations. This figure is adapted from Ref.
175 (CC BY 4.0).