Abstract
In recent decades, silicon photonics has attracted much attention in telecom and data-com areas. Constituted of high refractive-index contrast waveguides on silicon-on-insulator (SOI), a variety of integrated photonic passive and active devices have been implemented supported by excellent optical properties of silicon in the mid-infrared spectrum. The main advantage of the silicon photonics is the ability to use complementary metal oxide semiconductor (CMOS) process-compatible fabrication technologies, resulting in high-volume production at low cost. On the other hand, explosively growing traffic in the telecom, data center and high-performance computer demands the data flow to have high speed, wide bandwidth, low cost, and high energy-efficiency, as well as the photonics and electronics to be integrated for ultra-fast data transfer in networks. In practical applications, silicon photonics started with optical interconnect transceivers in the data-com first, and has been now extended to innovative applications such as multi-port optical switches in the telecom network node and integrated optical phased arrays (OPAs) in light detection and ranging (LiDAR). This paper overviews the progresses of silicon photonics from four points reflecting the recent advances mentioned above. CMOS-based silicon photonic platform technologies, applications to optical transceiver in the data-com network, applications to multi-port optical switches in the telecom network and applications to OPA in LiDAR system.Introduction
Silicon photonics is a contemporary favorite in recent photonic network. Originated from the strong demand of explosively expanding network traffic, optical interconnection in the telecom/data-com now inevitably requires the silicon photonic platform to have excellent optical properties of the silicon in the mid-infrared spectrum.
To begin with, what is the network traffic like? How did the network traffic among human beings progress in its history? A newspaper article1 (Fig. 1) provides a certain kind of answer: The ancient network traffic begins with mural painting in a cave (BC ~ 40, 000) and the birth of paper (AD 105). Through the appearances of movable type printing (~1450) and telephone (1876), the article takes three historical happenings, i.e., TV broadcasting (1936), Internet (1969) and World Wide Web (WWW) (1993) as the dawn of modern traffic in the telecom era. Highlight in the article is a forecast for the vast change of the global traffic volume per year from ~ 6 Exa-byte (6 × 1018 byte) in 2000 to ~ 40 Zetta-byte (~ 4 × 1022 byte) in 2020. Additionally, a future-forecast organization, Yole Développement, also reports similar data every year. The explosive growth involves the contributions of several communication systems and technologies in the optical telecom network, as shown in Fig. 2, since 1980s2. Representative innovative technologies include planar light-wave circuit (PLC), arrayed waveguide grating (AWG), optical fiber amplifier (OFA), dense wavelength division multiplexing (DWDM), re-configurable optical add-drop multiplexing (ROADM) and digital coherent technique.
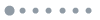
Figure 1.History of the traffic among human beings1.
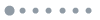
Figure 2.Progresses in the optical communication network2.
For the device technologies in 1980s, Ⅲ-Ⅴ compound semiconductor based laser, photo-detector and other active devices have played important roles in the progress of the photonic network. Ⅲ-Ⅴ based optoelectronic integrated circuit (OEIC) was first developed in 1980s. However, full scale of photonic device integration has started with the pioneering work on silicon photonics (hereafter referred to simply as Si photonics) by Soref and Bennett, in the late 1980s and early 1990s3. Initial motivation for works on the Si photonics is the compatibility with the mature complementary metal oxide semiconductor (CMOS) manufacturing with low-cost and mass-market applications. Another important motivation is the availability of high-quality Si-on-insulator (SOI) wafers as an ideal planar waveguide platform, capable of strong optical confinement based on the high index contrast between Si (n = 3.45) and SiO2 (n = 1.45). The latter motivation makes it possible to scale photonic devices down to hundreds of nanometer level. Such nano-scale dimensions are strongly required for true compatibility with large scale integration (LSI) processing.
Luxtera challenged pioneering integration of the photonic devices using the SOI CMOS process in 130 nm node4-6. Figure 3 shows the first demonstration of an optical grating connected to Si waveguides to show an efficient coupling (66 % at 1550 nm) of an optical single-mode fiber to nanoscale Si waveguides, thus mitigating the difficulty induced by the mode-size mismatch between them. This work has been widely recognized as the dawn of Si photonics, and they are now key devices for next-generation telecom and data-com interconnects for bringing the advantages of integrated photonics, i.e., high data densities and long transmission distances as well as the low manufacturing costs accompanied by CMOS compatibility.
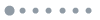
Figure 3.Demonstration of the first optical grating connected to silicon waveguides by Luxtera. Figure reproduced from ref.6, under a Creative Commons Attribution License.
For about ten years from early 2000 until middle 2010s, excellent reviews and roadmap on the Si photonics technologies have been reported5-11. These reviews have introduced a wide range of hybrid and monolithic integration of Si photonic active/passive elements and also early applications to telecom and data-com areas. Correspondingly, this paper quickly briefs the imprints of the Si photonic devices, then lays great emphasis on the recent applications such as Si photonic interconnect transceivers in the data-com system and Si photonic multi-port switches in the telecom network.
Section 2 surveys the progress of Si photonic platform technologies including CMOS process based heterogeneous integration of passive/active photonic elements. Section 3 reviews the remarkable progresses in two-decades of Si photonic transceivers applied to the data-com interconnect. Section 4 introduces the recent innovative activities on multi-port Si photonic switches for the ROADM and optical phased arrays for light detection and ranging (LiDAR) system. Section 5 summarizes these results together with challenges and perspectives of Si photonic platforms and future applications.
Progress of Si photonic platform
CMOS process-compatible Si photonics fabrication
Integration of photonic components into a single chip has long been expected for practical integrated optics. In the conventional silica-on-Si technology, the waveguide was formed in a silica layer with doped phosphor or germanium atoms12 or in a silicon oxy-nitride (SiON) layer13. On the other hand, SOI provides an excellent platform for Si/SiO2 waveguides caused by a large refractive index contrast between Si (n = 3.45) and SiO2 (n = 1.45), thus enabling strong optical confinement in the waveguides down-sized to approximately 0.1 μm2.
In 2004, Vlasov et al. demonstrated the Si photonic waveguide on the SOI substrate14. Owing to the small sidewall surface roughness using a standard CMOS fabrication line using 200 mm wafers, minimal propagation losses of 3.6 dB/cm for the TE polarization as well as the 90° bending losses of 0.086 dB and 0.013 dB for bending radii of 1 μm and 2 μm, respectively, were reported at 1.5 μm wavelength. Figures 4(a) to 4(c) show scanning electron microscope (SEM) images of a single-mode strip waveguide with 445 × 220 nm core cross-section at different orientations. Bending loss spectra with radii of 5 μm, 2 μm and 1 μm were also measured for TE and TM modes, as shown in Figs. 4(d) and 4(e). The TM mode in a small radius bend degrades the bending loss at longer wavelengths, while the TE mode shows almost flat loss spectra in less than 1550 nm wavelength region. These results realize ultra-dense photonic integrated circuits on a single Si chip.
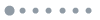
Figure 4.(a–c) SEM images of a single-mode strip waveguide cross-section at different orientations. (d, e) Bending loss spectra for TE and TM polarizations, respectively. Figure reprinted with permission from ref.14, Optical Society of America.
Heterogeneous integration of laser-on-Si platform
Integrated Si photonic platform includes on-chip active elements such as light source, modulator and detector as well as passive elements such as coupler, filter, grating and so on.
Among active Si photonic elements, Si nano-laser is difficult in principle because of inefficient radiative recombination due to its in-direct bandgap. In alternative direct bandgap Ⅲ-Ⅴ semi-conductors like gallium arsenide (GaAs), the bottom of the conduction band and the top of the valence band coincide in the wave vector axis, thus enabling efficient radiative recombination. Nevertheless, Si exhibits many excellent properties: (i) extremely high purity and low defect density, (ii) availability of the state-of-the-art CMOS technology, (iii) high thermal conductivity valuable for an active device substrate. These advantages allow for low-loss Si waveguides, one order of magnitude lower than the compound semiconductor waveguides. Therefore, an electrically pumped efficient Si nano-laser has become a long-term challenge for making the most of aforementioned Si advantages. For this purpose, direct mount of Ⅲ-Ⅴ lasers on Si is a promising solution for on-chip semiconductor laser10. The first method of this type is chip-/wafer-bonding of the Ⅲ-Ⅴ laser on Si15, 16. The second one is direct epitaxial growth of the Ⅲ-Ⅴ layer on Si or SOI supported by intermediate buffer layers such as germanium layer and strained super-lattices17, 18. The third one is a combination of these approaches. Next, the first two approaches are overviewed.
Chip-/wafer-bonded Ⅲ/Ⅴ-on-Si laser
A chip-bonded hybrid Ⅲ-Ⅴ-on-Si laser has the advantage of minimizing the area of the Ⅲ-Ⅴ material15 when compared to that of wafer bonding to form the SOI based Si photonic platform that has been demonstrated so far16, 19, 20. The wafer-bonding technology has the advantages over chip-bonded Ⅲ-Ⅴ laser while on an SOI host substrate. In the wafer-bonding, the Si passive light-wave circuits are patterned before the bonding process and the aluminum-gallium-indium arsenide (AlGaInAs) layers are processed after bonding using the standard techniques to fabricate Ⅲ-Ⅴ lasers21. After laser excitation, optical field is confined mainly in the Si waveguide with a fraction contained in the Ⅲ-Ⅴ quantum wells. The confinement factor can be significantly changed by altering the waveguide width. The advantage of this structure is that the optical mode can obtain electrically pumped gain from the Ⅲ-Ⅴ region, while being guided by the underlying Si waveguide region22.
The vertical cavity surface emitting laser (VCSEL) is another promising light source for Si photonic circuits because of its small footprint, low manufacturing cost, low power consumption, capability of two-dimensional (2D) array and high data transmission rates23-25. Recently, an electrically pumped AlGaInAs-Si VCSEL with a high index-contrast grating (HCG) mirror on an SOI substrate has been reported. The HCG provides > 95% coupling efficiency between a surface-normal input and an in-plane SOI waveguide26-27. Figure 5(a) shows a schematic of the HCG VCSEL using an ultra-thin Si HCG optimized substrate as a bottom reflector in the laser cavity on a SOI substrate. The active layer AlGaInAs consists of compressively-strained quantum wells, and employs a proton implant defined aperture for current confinement, as illustrated in Fig. 5(b). The laser cavity, indicated by circulating red arrows in Fig. 5(a), is formed by the Ⅲ-Ⅴ distributed Bragg reflector (DBR) top mirror and the Si-HCG bottom mirror. The VCSEL operates at near infrared (IR) wavelengths with > 1 mW continuous wave (CW) power. The VCSEL is also capable of > 2.5 GHz 3-dB direct modulation bandwidth. These results present a promising approach for scalable and low cost integrated photonic circuits including active components.
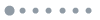
Figure 5.Schematic of VCSEL with Si HCG as bottom mirror.
(a) Tilted-view of VCSEL cross-section with circulating red arrows indicating optical cavity. (b) The VCSEL employs a proton implant-defined aperture for current confinement, indicated by red curved lines between contacts. Figure reprinted with permission from ref.27, Optical Society of America.
Epitaxial-grown Ⅲ-Ⅴ on-Ge/Si laser
In general, direct epitaxial growth of Ⅲ-Ⅴ compounds on Si substrates has considerable difficulty due to large lattice mismatch and different thermal expansion between Ⅲ-Ⅴ and Si. In contrast, Ge-on-Si (Ge/Si) has become a favorable technology for mitigating the lattice mismatch between GaAs and Si28.
Recently, InAs/GaAs quantum dots (QDs) have been grown successfully on Ge/Si substrates29. Using this technology, the first CW operation of on-Si InAs/GaAs QD laser at room-temperature (RT) has been reported, as shown in Fig. 6. A Ge layer was grown on a GaAs buffer layer, resulting in the high-performance Ⅲ-Ⅴ QD laser monolithically grown on the Ge substrate30, 31. Characteristics of the first RT CW operation of Si-based QD laser were Jth of 163 A/cm2 at a wavelength of 1.28 μm using 20 μm-wide, 3~3.5 mm-long cavities.
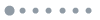
Figure 6.Schematic layer structure of an InAs/GaAs QD laser on a Ge-on-Si substrate. Figure reprinted with permission from ref.31, Optical Society of America.
Heterogeneously integrated narrow-linewidth laser
Recent progress of optical communications with high speed and wide band-width requires narrow linewidth lasers for more complex modulation methods. For example, a tunable laser with a linewidth < 300 kHz is needed for a 16-quadrature amplitude modulation (QAM) format32. Komljenovic et al. have demonstrated a widely tunable narrow-linewidth semiconductor laser with monolithically integrated on-chip cavity using a Si photonics platform on an SOI substrate33. The gain section is sandwiched by loop-mirrors, constituting a 2 mm long cavity. The lasing wavelength is determined in the tuning section comprised of two ring resonators and a cavity phase section, all of which are controlled by thermal phase tuners. The external cavity is ~ 4 cm long and has its own phase adjustment and gain section. The external cavity is coupled on the side with the tuning section so that the tuning rings filter out any spontaneously emitted light from the extended cavity. The lasers are tunable over a 54 nm range from 1237.7 to 1292.4 nm. The measured linewidth in full tuning range is below 100 kHz and even ~ 50 kHz, a new record for narrow linewidth in a monolithically integrated widely-tunable laser design.
Integration of passive Si photonic platform
So far, on-Si passive photonic elements such as waveguides, couplers, multiplexers, polarization controllers, filters, resonators, etc. have been implemented14, 34. Figures 7 show several SEM photographs of compact Si/SiO2 waveguides. Figures 7(a) and 7(b) show multiply coupled micro-rings and their magnified ones with radii of only 1 μm, while Fig. 7(c) shows sharp-tapered waveguide. Their bending and propagation losses are less than 0.09 dB and 1 dB/cm, respectively10, 35, 36. Reference37 also reports schematic design of multi-octave spectral beam combiner with integrated lasers utilizing Si and Si3N4 waveguides.
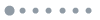
Figure 7.SEM photographs of nano-scale Si/SiO2 waveguides.
(a, b) Multiply coupled micro-rings and their magnified ones. (c) Sharp taper. Figure reprinted with permission from ref.10, SPIE.
In the WDM system, an AWG is an essential optical component for multiplexing channels of several wavelengths into a single optical waveguide at the transmission end or de-multiplexing individual channels of different wavelengths at the receiving end. Figure 8(a) shows a schematic for the operating principle of the AWG. An optical signal consisting of multiple wavelengths in the input waveguide is diverged in an expanding free-propagation region (FPR) as an input star coupler. An array of waveguides (designed as a phased array) captures this diverging light, which then propagates and is focused at the end, i.e., at the input aperture of the output focusing FPR. This is because the length of array waveguides is selected so that the optical path length difference between adjacent waveguides equals an integer multiple of the central wavelength of the de-multiplexing. As a result, the field distribution at the beginning of the phased array is reproduced at the end and, in the focusing FPR, the light beam interferes constructively and converges at one single focal point on the focal line, as shown in the Fig. 8(a). If the wavelength is shifted from the central wavelength, the light beam is focused on a different position of the focal line in the image plane. Thus the field for each wavelength can be coupled into the respective output waveguide. As an extremely compact AWG chip demonstrated by Yaegashi's group38, Fig. 8(b) shows a photomicrograph of the AWG with about 1.4 mm square, separating up to eight wavelengths at an optical frequency interval of 100 GHz. Figure 8(c) shows the filter spectral characteristics, indicating a low 1.2 dB loss and -16 dB crosstalk between wavelengths.
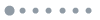
Figure 8.(a) Schematic showing the operating principle of the AWG. (b) Photomicrograph of the AWG with about 1.4 mm square and eight wavelength channels. (c) Filter spectral characteristics. Figure reprinted with permission from: (b, c) ref.38, OKI Corporate.
Another important optical component for the WDM system includes an optical-cavity based micro-ring resonator (MRR) filter with periodical Lorentzian spectral responses to select a series of resonance wavelengths. In general, the cavity should have a large free spectral range (FSR) to cover all the multiplexed channels. Many cascaded MRRs are convenient for an array with N × N elements to deal with N channels39. In general, a large wavelength window might be required for course WDM (CWDM) on-chip optical interconnects, and thus it is preferable to use MRR filters with an ultra-large FSR and a large tunable range of wavelength. Dai et al demonstrated the SOI-nanowire based MRR, in which the FSR increases from 17 nm to 58 nm for the bending radius reduced from R = 5 μm to R = 1.5 μm. When MRRs are used as tunable optical filters, modulators, switches, etc, the size reduction is effective for reducing the power consumption40.
Growing Si photonic foundries
Integrated Si photonic devices are produced by several manufacturing steps such as design, CMOS-process fabrication, packaging and testing by each special company (supply-chain). Table 1 shows the 2019 world ranking of semiconductor companies reported by marketing company Yole Développement41. In the table, a fabless company is the one that designs and sells hardware and semiconductor chips but outsources the fabrication of such chips and hardware, while a (pure-play) foundry (or just fab) is a factory that only manufactures devices such as integrated circuits without designing them. In late 2000s through early 2010s, the main Si photonics manufacturing foundries were represented by Luxtera, Intel, Cisco, etc. In "Zero change" monolithic CMOS-processed Si photonic transceiver Section, the new foundry, GlobalFoundry (GF), will be introduced as a typical foundry that manufactured "Zero change" monolithic CMOS-processed Si photonic transceivers. GF is the world's second-largest pure-play foundry with 2017 revenues estimated at $5.86 billion by market research company IC Insights, as shown in Table 242. Since the function and scale of Si photonic platform have become more complicated and expanded year by year, the system of this production division and the role of the foundry will become more indispensable, as suggested in Table 242.
Table 1. Silicon photonics supply chain for optical transceivers41. (Source: Silicon Photonics report, Yole Développement, 2020)
2017rank | 2016rank | Company | Foundrytype | Location | 2015 sales($M) | 2016 sales($M) | 2016/2015change (%) | 2017 sales($M) | 2017/2016change (%) |
*Includes Huahong Grace and Shanghai Huali.Source: IC Insights company reports |
1 | 1 | TSMC | Pure-Play | Taiwan, China | 26, 574 | 29, 488 | 11 | 32, 163 | 9 |
2 | 2 | GlobalFoundries | Pure-Play | U.S. | 5, 019 | 5, 495 | 9 | 6, 060 | 10 |
3 | 3 | UMC | Pure-Play | Taiwan, China | 4, 464 | 4, 582 | 3 | 4, 898 | 7 |
4 | 4 | Samsung | IDM | South Korea | 2, 670 | 4, 410 | 65 | 4, 600 | 4 |
5 | 5 | SMIC | Pure-Play | China | 2, 236 | 2, 914 | 30 | 3, 101 | 6 |
6 | 6 | Powerchip | Pure-Play | Taiwan, China | 1, 268 | 1, 275 | 1 | 1, 498 | 17 |
7 | 8 | Huahong Group* | Pure-Play | China | 971 | 1, 184 | 22 | 1, 395 | 18 |
8 | 7 | Tower Jazz | Pure-Play | Israel | 961 | 1, 250 | 30 | 1, 388 | 11 |
― | ― | Top & Total | ― | ― | 44, 163 | 50, 598 | 15 | 55, 103 | 9 |
― | ― | Top & Share | ― | ― | 87% | 88% | ― | 88% | ― |
― | ― | Other Foundry | ― | ― | 6, 597 | 7, 112 | 8 | 7, 207 | 1 |
― | ― | Total Foundry | ― | ― | 50, 760 | 57, 710 | 14 | 62, 310 | 8 |
Table 2. Major 2017 foundries (Pure-Play and IDM (integrated device manufacturer)). Table reprinted with permission from ref.42.
Si photonic interconnect in data center and HPC
Pioneering Si photonic transceiver
Si photonics includes integrated optical and electronic components on single chip fabricated with a standard CMOS process. In the current interconnect in the data center and high-performance computer (HPC), the considerable amount of copper wires which connect different electronic components degrade the signal intensity due to the electrical resistance in the wire and have a limited maximum length. One possible solution for higher data rates over long distances is to replace electrical interconnection with an optical link, i.e., optical interconnection based on Si photonic transceivers. Figure 9 shows a general architecture of the Si photonic transceiver composed of a transmitter and a receiver as key elements of an optical link. The transmitter is constituted of an on-chip laser source, optical modulators and Si waveguides, while the receiver is constituted of on-chip photodetectors and electronics converting the data into electronic signals. Since the figure shows a four-channel WDM optical link, the transmitter output involves a multiplexer which combines four different-wavelength light beams and send them into a single interconnect waveguide, while the receiver input involves a de-multiplexer which separates and sends them into four different detectors.
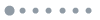
Figure 9.Basic block diagram of an optical link including a transmitter and receiver.
A pioneering Si photonic transceiver with optical fibers was demonstrated in 2007 by Intel group, as shown in Fig. 107, 43. The figure explains a 50 Gb/s optical link module. In Fig. 10(a), a transmitter chip with four hybrid InP/Si lasers emits four different wavelength CW laser beams, which propagate into 12.5 Gb/s-encoded optical modulators. These four beams are combined in a multiplexer and sent into a single optical fiber for a total data rate of 50 Gb/s. At a receiver, as shown in Fig. 10(b), the chip separates four light beams in the de-multiplexer and sends them into photodetectors. Development of the chip achieving data rate of 100 Gb/s or further is going on.
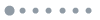
Figure 10.50 Gb/s Si photonics transmit module.
(a) and (b) are transmitter and receiver, respectively, with wavelength division multiplexing. Figure reproduced from ref.43, Intel.
Luxtera, as shown in Fig. 3 as a pioneering Si photonic platform company, reported a 10 Gb/s transceiver with a four-channel WDM transmitter/ receiver in 20104. It was fabricated with a 90 nm SOI CMOS process. The chip includes monolithically integrated wavelength filters, photodetectors, electronic amplifiers and drivers except for four InP lasers which are flip-chip bonded onto the Si substrate. As shown later, these kinds of on-chip Si photonic transceiver have been most widely developed by several companies and foundries,
Progress of Si photonic transceiver
Si photonic interconnect forecast
Si photonics is now widely accepted as a key technology in the next-generation telecom and data-com systems. A. Zilkie overviews9 that the potential of integrated Si photonics was recognized in the first studies of SOI waveguides in 198544, 45, and their commercialization started in 1989 by Bookham Technology Ltd46. Practical applications of Si photonics first started in sensor areas in the 1990s and afterwards their commercialization changed to WDM telecomm products. The practicality for data-com market was recognized further with the realization of SOI-waveguide p-i-n junction modulators45 and Ge- and SiGe-based photodetectors and modulators47, 48. Pioneering Si photonics companies such as Luxtera49, Kotura (now Mellanox)48 and Acacia Communications50 are producing Si photonic 100 Gb/s transceivers for data center/HPC interconnects and telecom optical transport markets. Figure 11 shows Si photonics 2019–2025e forecast in the optical data center market, where the mega data center/HPC companies are planning for large-scale deployment of Si photonics based interconnects in the hardware replacement cycles starting in ~201951. Metrics in ref.51 indicated that the increase of the adoption in the data center and other data-com markets results in low cost per data lane (~$1/Gb/s), low power consumption per data lane (few pJ/bit), and good manufacturability and reliability.
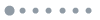
Figure 11.Silicon photonics shipments, for datacenter (in units) 2019-2025e.
(Source: Silicon Photonics report, Yole Développement, 2020)
Taking note to the process integration and its future evolution, Boeuf reviews in ref.9 that the evolution of Si photonics in the next decade will require the implementation of several new process steps. He mentions that an efficient device optimization can be first achieved from a front-end perspective by mixing several types of waveguides like strip and rib waveguides with various SOI thicknesses. New material layers are integrated for mitigating the intrinsic weaknesses of Si such as thermal sensitivity of the refractive index and indirect-band-gap originated inefficient radiation. "Midex" materials having refractive index between ~1.6 to 2.5 and able to mitigate Si thermal properties issues will be developed. Regarding the integrated light source, the III–V/Si hybrid laser has already been reviewed in Chip-/wafer-bonded Ⅲ/Ⅴ-on-Si laser Section. Nevertheless, the monolithic integration of the hybrid laser into the Si photonics transceiver will require further innovative developments. From the interconnect perspective, on the other hand, development of through-Si-via (TSV) will enable the Si photonics interposer technology which allows for the integration of complex electronics together with the driving electronics of the photonics.
In the next part, advanced approaches of IBM toward Si photonic multi-chip-module are reviewed.
IBM roadmap on Si photonic multi-chip-module
Initially, Si photonic transceivers have mainly progressed in the data center and HPC areas. Within the data center, transceivers are located at the edge of the packaging board in each server, resulting in large distances between the optical component and the processor chip. IBM has been developing packaging designs to solve the issue for fifteen years under the strategy of Si photonic die to be integrated directly into the processor module. Patterson et al. have reviewed the IBM strategy of Si photonic multi-chip-module (MCM) and the first CMOS integrated Si photonics chip demonstrated in 201552. Figure 12(a) shows a Si photonic die designed to arrange a waveguide array for efficient coupling between light wave and electronic components via an array of flip-chip bumps to support electrical signals. Laser dies are attached to the Si photonic chip through flip-chip bumps. Figure 12(b) shows a ball grid array (BGA) substrate with a central logic die, two hybrid memory cubes (HMCs) and associated CMOS devices. Alternative designs are illustrated in Figs. 12(c) and 12(d). In these cases, the logic die and high-bandwidth memory (HBM) stacks are mounted directly onto a high-density interposer to link the logic, memory and photonic integrated circuit closely.
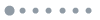
Figure 12.Si photonic MCM.
(a) Photonic integrated circuit (PIC) with flip-chip mounted laser die and edge-coupled waveguide. (b) Logic, HMC and PIC mounted directly to a BGA substrate. (c) Logic and HBM mounted on a high-density interposer with micro-bumps. (d) Cut-away revealing copper pillar, flip-chip, BGA and optoelectronic interconnections. Figure reprinted with permission from ref.52, IBM.
Figure 13(a) shows a photograph of the first CMOS integrated CWDM Si photonics chip demonstrated in 2015. The chip is capable of transmitting and receiving four-wavelength channels, each operating at 25 Gb/s on a single die. The die was manufactured by 90 nm CMOS process technology, containing modulators, Ge photodetectors, and ultra-compact WDMs. Figure 13(b) shows a die with an array of 100 μm lead (Pb)-free flip-chip bumps on a 200 μm pitch. Figure 13(c) shows transmitter and receiver elements along with their eye diagrams, indicating a clean and high quality response. The lasers are connected off-chip with the optical signals. Four wavelength signals are multiplexed into 100 Gb/s fiber ports. The updated chip design allows for up to twelve such fiber ports, thus enabling 600 Gb/s bi-directional data rates from a single optical transceiver. The demonstration was to push 100 Gb/s data speeds over a range of up to 2 km as required by large data center environments53.
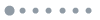
Figure 13.IBM Si CWDM 4 × 25 Gb/s die.
(a) Photograph of CMOS integrated Si photonics chip. (b) Die with an array of 100 μm Pb-free flip-chip bumps on a 200 μm pitch. Two optical I/Os are seen at the bottom of the die. (c) Transmitter and receiver elements along with their eye diagrams Figure reprinted with permission from ref.52, IBM.
In recent years, two innovative progresses on the new types of Si photonic transceiver technologies have been reported: One is a "Zero change" monolithic CMOS processed Si photonic transceiver and the other is a fingertip-size Si photonic interconnect for ultra-short optical links. Their characteristics are reviewed in the following.
"Zero change" monolithic CMOS-processed Si photonic transceiver
In 2018, Stojanov et al. reported the most advanced monolithic Si photonic platforms in the state-of-the-art SOI CMOS processes as the world's first micro-processor with photonic I/O11. They realized record high energy-efficiency/speed in transmitters as well as the highest level electronic-photonic integration. It is called the "Zero-change" Si photonic platform implemented using an unchanged commercial 45 nm CMOS SOI process54. As shown in Fig. 14, all photonic devices are designed to follow the purely-electrical foundry design flow without any modifications to the native process11, 55, 56. Optical devices are implemented in the sub 100 nm thick high-index crystalline Si (c-Si) layer. The first six metal layers on top of photonics structures have been eliminated to prevent metallic optical losses. Since the buried oxide (BOX) layer is thin, the Si substrate is removed also to reduce the waveguide optical loss to approximately 3 dB/cm in it55. Light is coupled to the chip via vertical grating couplers. Active devices including micro-ring modulators and photodiodes are implemented using existing source/drain and well-implanted doping levels as well as the available SiGe in this process.
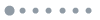
Figure 14.Schematic cross-section of 45 nm SOI CMOS process based Si photonic platform with photonic as well as electronic devices at the front end and bump at the back end. Figure reprinted with permission from ref.56, IEEE.
Figure 15 presents the demonstrated processor with photonic I/O using the 45 nm "Zero-change" platform. Ultra-power-efficient ring resonator (RR) based Si photonic links, with millions of transistors and hundreds of photonic devices fabricated on the same chip, are aimed to improve processor-memory link bandwidth11, 56. The system-on-chip (SoC) in Fig. 15(a) has a dual-core reduced instruction set computer-V (RISC-V) processor11, 57, 1 MB static random access memory (SRAM) based cache memory, and DWDM optical I/Os, as illustrated in Fig. 15(b). Figure 15(c) shows the key photonic devices of an optical link implemented by this technology.
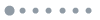
Figure 15.Photograph of "Zero-change" SOI platform die.
(a) SoC including a RISC-V processor, SRAM based cache memory and DWDM optical I/Os. (b) WDM transceivers. (c) Key photonic devices of an optical link. Figure reprinted with permission from ref.11, Optical Society of America. Development timeline in Fig. 3, ref.11 has been omitted here.
For passive optical elements, waveguides are built in the sub-100 nm thick c-Si body layer by blocking all transistor body dopants to lower the optical loss. In the 45 nm platform, the measured loss is 3.7 dB at 1280 nm and 4.6 dB at 1550 nm55. For coupling between the on-chip waveguide and optical fiber, vertical grating couplers have been developed using two layers of c-Si and transistor gate poly-Si for designing uni-directional grating couplers. 1.5 dB optical loss has been achieved58.
For active optical elements, micro-ring modulators (MRM) have been fabricated by placing interleaved p and n junctions along the ring cavity, as shown right in Fig. 15(c). Resonance wavelength can be modulated by changing the carrier density in the depletion regions of interdigitated junctions via carrier-plasma effect in silicon3. 5 μm-radius active micro-rings achieved intrinsic Q-factors of 18 k and up to 10 k loaded Q-factors with 3.2 THz FSR in telecom O-band11. For photodetectors fabricated in the die, refer to ref.11 for details.
As compared with the well-known Mach-Zehnder modulator (MZM) with mm size, the MRM with compact footprints fits for high-speed and energy-efficient optical transmitters, so that it enables DWDM for large-scale integrated systems with high bandwidth densities in the Tb/(s∙mm2) range. Experimental results using the MRM-based transmitters show two orders of magnitude improvement in energy efficiency as compared with currently popular MZM based transmitters. Figure 16(a) shows a photograph of a 40 Gb/s non-return-to-zero (NRZ) transmitter die in the 45 nm SOI CMOS. The modulator and driver stage consume only 40 fJ/b and the total energy consumption and area are 0.7 pJ/b and 0.03 mm2, respectively11. Figure 16(b) shows the measured NRZ eye diagrams at 40 Gb/s with the extinction ratio of 3 dB and insertion loss of 4.7 dB. For data-rates higher than the modulator bandwidth, 40 Gb/s pulse amplitude modulation (PAM)-4 transmitter has been demonstrated11, 59. MR based optical transceivers can be used to build DWDM links to achieve Tb/s aggregate bandwidths over a single fiber. The latest performance and results of the "Zero-change" Si photonics platforms presented above indicate that the 45-32 nm SOI CMOS processes provide a "sweet-spot" for adding photonic capability and enhancing integrated system applications beyond the Moore-scaling11, 59.
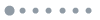
Figure 16.40 Gb/s transmitters.
(a) Micrograph of the 40 Gb/s NRZ transmitter. (b) NRZ transmission eye-diagram. Figure reprinted with permission from ref.11, Optical Society of America. Figs. 7(b) and 7(d) in ref.11 have been omitted here.
Fingertip-size Si photonic interconnect for ultra-short optical links
Recently, field-programmable gate arrays (FPGAs), having a large input/output (I/O) bandwidth over 1 Tb/s, are attracting much attention, in particular, in the data center and HPC for supplementing the deficient processing speed of current central processing units (CPUs) and/or graphic processing units (GPUs). To satisfy these bandwidth requirements, a small footprint optical module with high bandwidth density, low power consumption and high temperature durability is required to be mounted around the FPGA. To solve this problem, a Si photonics based fingertip-size optical module named "optical I/O core" has been developed recently by the PETRA project. They demonstrated 300 m transmission at 25 Gb/s per channel with the FPGA board mounted by the optical I/O cores60, 61.
Figure 17(a) shows a whole part of the optical I/O core chip and layout of optical and electrical I/O's as well as integrated circuit mounting areas, respectively61. It has a small footprint of 5 mm × 5 mm and a maximum capacity of 300 Gb/s (25 Gb/s × 12 channels).
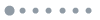
Figure 17.(a) Whole part of optical I/O core chip. (b, c) Cross-sectional views of receiver and transmitter, respectively. Figure reprinted with permission from ref.61. AIOCORE.
Figures 17(b) and 17(c) show cross-sectional pictures of a receiver and a transmitter, respectively. The transmitter includes optical waveguides, MOS-capacitor type Si optical Mach-Zehnder interferometer (MZI) modulator and grating coupler (GC), while the receiver includes a Ge-photodetector (PD) as well as waveguides. Si photonics constituting the core is fabricated using a 300 mm SOI wafer process. ArF-immersion lithography enables low-loss (1.28 dB/cm for the O-band) and high-uniformity optical waveguide62.
After completing the Si photonics integrated circuit, a Fabry-Perot laser diode (FP-LD) and optical pins were assembled into the optical I/O core. Figure 18 shows a cross-sectional view of the Si photonics platform in part on which the FP-LD is assembled60. By means of designing alignment marks on the LD and Si platform, horizontal and vertical misalignments between the LD and the Si platform remained ± 0.5 µm and ±0.1 µm, respectively. For selecting the LD on Si photonics, InAs/GaAs quantum dot (QD) FP-LDs were evaluated to be superior to InGaAsP quantum well FP-LDs in high temperature operation and high optical feedback tolerance.
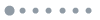
Figure 18.Cross-sectional view of Si photonics integrated circuit with FP-LD. Figure reprinted with permission from ref.60, The Institute of Electronics, Information and Communication Engineers.
Another important optical component in the optical I/O core is a newly developed optical pin, as shown in Figs. 17(c) and 17(d). The optical pin is a 3D resin polymer waveguide used in place of an optical lens for connecting the grating coupler or the Ge-PD with multi- mode fiber (MMF). Since the FP-LD is not temperature-controlled, the output wavelength from the core is seriously temperature-dependent (0.6 nm/℃) and the radiation angle from the grating coupler is also fluctuated at 0.083 degree/nm. Nevertheless, the optical pin has a high refractive index contrast between the core and cladding, so that the varied radiated light from the grating coupler can be enclosed within the core63. This means that the calculated optical pin-GI50 MMF misalignment tolerance with 1 dB excess loss is larger than 10 µm in a temperature range from -45 ℃ to +85 ℃. The result significantly contributes to the excellent overall characteristics of the optical I/O core, as shown later. It also provides an easy connection between the optical I/O core and the MMF.
Before mounting the optical I/O cores around the FPGAs, temperature dependence of eye patterns and bit error rates (BERs) at 25 Gb/s of the optical I/O core were evaluated. Clear eye patterns from 25 ℃ up to 85 ℃ and BERs less than 10-12 at 20 ℃ and 85 ℃ were demonstrated64. These results were achieved owing to the QD LD and optical pin technologies. The transmission characteristics of the optical I/O core were also evaluated using two types of MMFs, as shown in Fig. 19. One is a corning clear curve LX MMF65 specialized for the O-bands. The other is a conventional OM3 fiber used in 850 nm wavelength. The former MMF enables transmission up to 300 m at 25 Gb/s sufficient for applications in the data center system, while the latter OM3 fiber enables 30 m transmission without an equalizer or 60 m transmission with an equalizer at 25 Gb/s sufficient for applications in the HPC system. Finally, the performance of the optical I/O core module mounted around the FPGA board was evaluated. On the board, maximum 24 transceiver and receiver channels of optical I/O cores with a capacity of 1.2 Tb/s were mounted around FPGA. The FPGA boards were connected to the 300 m MMF and 25 Gb/s signals per channel were transmitted. BERs less than 10-12 with good eye patterns were successfully obtained. The result shows that almost no degradation occurs in BERs at the simultaneous many-channel operation in the optical I/O core.
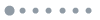
Figure 19.Transmission characteristics of the optical I/O core with two kinds of MMFs. Figure reprinted with permission from ref.60, The Institute of Electronics, Information and Communication Engineers.
In conclusion, the Si photonics based fingertip-size optical I/O core with features of high bandwidth density, low power consumption and high temperature durability allows for 300 m transmission at 25 Gb/s per channel with the modules mounted around FPGA. These results will contribute to high bandwidth density and low power consumption interconnects among FPGAs in data center and HPC systems60.
Si photonic switch for innovative applications
Architecture of photonic switches
Optical switches are essential elements for constructing integrated Si photonic circuits in the photonic network. For designing integrated multi-port optical switches, physical mechanisms of the switch and topologies of the switch fabrics should be considered. Typical switching mechanisms include thermally and electrically operated phase modulation in inter-ferometric structures like Mach-Zehnder interferometer (MZI) and micro-ring resonator (MRR), and signal amplification or absorption in semiconductor optical amplifiers (SOAs)66. They will be discussed later and here, typical switch fabric topologies (with N × N port counts) are introduced. Metrics of the performance in each topology are blocking characteristics, crosstalk suppression, number of cascading stages and total number of switch cells. Commonly used topologies include crossbar, path-independent-loss (PILOSS), Beneš and switch-and-select (S & S), as shown in Fig. 20, all of which support non-blocking connections67. The crossbar topology has the most cascading stage number, resulting in a large path-dependent loss. The PILOSS is preferred when uniform performances across all paths are necessary. While the first-order crosstalk is inevitable, although not all the switch cells carry two signals at once68. The Beneš architecture has the smallest number of cascading stages as well as switching cells, while it suffers from situation of two traversing signals, resulting in the first-order crosstalk. The S & S topology blocks first-order crosstalk, but it requires the largest number of switch cells. Moreover, the central shuffle network becomes complex. The selection of topology depends on the switching performance.
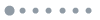
Figure 20.Schematic of typical optical switch topologies.
(a) Crossbar. (b) PILOSS. (c) Beneš. (d) Switch-and-select. Figure reprinted with permission from ref.66, Optical Society of America.
Si photonic switch in telecom network
Architecture of CDC-ROADM system
In the recent photonic network, re-configurable optical add-drop multiplexing (ROADM) has brought new flexibility and scalability to the conventional static photonic transport networks69. Multi-degree ROADM was developed after the introduction of the basic 2-degree ROADM in order to realize mesh-based network topologies70.
Figures 21(a) and 21(b) show examples of multi-ring network and 4-degree ROADM node configurations connecting two ring networks, respectively. Incoming WDM signals from the degree-1 input fiber are delivered to the drop-side optical switch. In Fig. 21(b), the optical switch selects the optical path to degree 2 (red), degree 3 (orange), or degree 4 (green) for each wavelength signal. When transponders (upon receiving signals, emitting different signals in response) at this node receive these signals, the optical switch selects the drop path (purple) to deliver the signals to another optical switch, namely a transponder aggregator (TPA). Then, the TPA allocates the input signals to each desired transponder for each wavelength channel. On the add-side, an output signal from the transponder is routed to an add-side optical switch via an add-side TPA. The add-side optical switch collects add signals and the signals that pass through this node are then launches them into the output fiber. Today, in addition to the multi-degree function, colorless, directionless, and contention-less (CDC) functions are expected to play important roles in achieving more flexibility in terms of wavelength routing and wavelength assignment71. Today, CDC-ROADM is considered to construct cost-effective photonics transport networks in long-haul and metro area transmission systems. Each function in the CDC-ROADM is shown next.
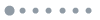
Figure 21.(a) Example of multi-ring network. (b) 4-degree ROADM node configuration connecting two ring networks. Figure reprinted with permission from ref.69, NTT Technical Review.
Multi-cast Si photonic switch
Explosively growing network traffic of the recent time accelerates the improvement of flexibilities72 and the evolution of the CDC-ROADM technologies70, 73 along with the adaptive digital coherent technologies74. In 2010's, Nakamura et al. have developed multi-cast Si photonic switches for ROADM applications75. Figure 23(a) shows two cases for reconfiguring optical paths in CDC-ROADM networks. When an optical path originally set between the nodes A and B is cut accidentally, the CDC-ROADM function smoothly offers an alternative optical path as an efficient failure recovery. Or, if an optical path originally set between the nodes C and D is forced to change into the path between the nodes C and E due to a rapid traffic accidental change, the CDC-ROADM function offers an optical path change as a prompt optical path reallocation. Nakamura et al. proposed optical switching in the CDC-ROADM both in the wavelength cross-connect and the TPA parts, as shown in Fig. 23(b). Compact and port count extendable TPA improves the flexibility of photonic networks. A cost-effective way for obtaining such TPA is by the combination of multi-cast optical switches and optical amplifiers73, 76–78. In the multi-cast switches, splitters enable optical paths with different wavelengths between one transmission line and multiple transponders, while filters, or local oscillators for digital coherent receiver enable selection of a wavelength channel on the receiver side. Here, a compact 8 × 8 multi-cast switch as a unit has been used, as shown in Fig. 23(c). The port count on the transponder side can be extended by adding these switch modules and external splitters. As shown in Fig. 23(d), eight 1 × 8 splitters and eight 1 × 8 selectors are connected in a full mesh manner. As shown in Fig. 20 previously, there are various configurations for point-to-point or unicast connection between multiple input and output ports such as PILOSS, cross-point type and Beneš type. These configurations need additional complicated control on elements when the multi-cast connection is introduced. However, the configuration in Fig. 23(d) can provide strictly non-blocking and multicast capabilities. In general, these structures should solve several problems for minimizing the excess loss of the optical switch module, achieving low polarization-dependent loss (PDL) characteristic and faster switching time (e.g., ~ 1 ms). Nakamura et al solved these problems by using Si integrated small-chip based 152 thermo-optic (TO) MZ optical switch elements, as follows.
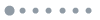
Figure 23.(a) Two cases of reconfiguring optical paths in photonic networks with CDC-ROADMs. (b) Schematic of CDC-ROADM. (c) TPA consisting of multiple optical switch modules. (d) Configuration of 8 × 8 multi-cast optical switch. Figure reprinted with permission from ref.75, IEEE.
Si photonic switch in the data center network
In the data-com networks, dynamic optical path switching is a promising method to reduce the power consumption similar to the telecom networks80. The key device is a low cost, high port-count and strictly non-blocking optical switch with high-density photonic integration using a CMOS-compatible process on large wafers81-83. Cheng et al. have recently discussed photonic switching in high performance data centers. They addressed thermo-optics (TO) and electro-optics (EO) from the view-point of switching effect, as well as MZI and MRR from the view-point device structure66. Here recent demonstration on the TO based multi-port MZI Si photonic switch by Tanizawa et al84 and a wide range of reviews on the EO based multi-port MZI and MRR photonic switches by Cheng et al66 are shown.
TO based multi-port MZI Si photonic switch
Recently Suzuki et al. reported a compact 8 × 8 strictly non-blocking TO switch based on Si-wire waveguides83, while Tanizawa et al. reported an ultra-compact 32 × 32 strictly non-blocking Si wire optical switch with the fan-out land grid array (LGA) interposer84. The switch employs a PILOSS topology85 and ensures port-count scalability in terms of power consumption since only N element switches should be active in an N × N switch. Figure 26 shows a top view of the 32 × 32 switch chip. It involves integrated 1024 2 × 2 MZ element switches, 961 intersections, 11 mm × 25 mm footprint die and electrode pads for flip-chip connections. The switch is 46 times as small as the 115 mm × 110 mm silica-based 32 × 32 PILOSS switch86. The two technical challenges of high intra-die uniformity and high-density electronic packaging have been solved.
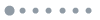
Figure 26.32 × 32 Si TO MZI-based PILOSS switch. Figure reprinted with permission from ref.84, Optical Society of America.
The MZ switch consists of two 3 dB directional couplers for the TM mode and TO phase shifters with a TiN heater on both arms. Si waveguides are 430 nm wide and 220 nm high, and buried by 1.5 μm-thick SiO2 cladding. The MZ element switch is designed to be in the cross state without heating. The 32 × 32 switch was fabricated using facilities for prototyping 45 nm CMOS transistors on 300 mm wafers. The footprint of the switch including the LGA interposer is 36 mm × 25 mm. Transmission characteristics of the 32 × 32 switch with LGA interposer were measured. Any path contains 32 MZ element switches and 31 intersections in the PILOSS topology. Six paths 1-12, 1-20, 1-32, 32-1, 32-13, and 32-19 were selected to show typical performances, in which 96 MZ element switches in total operate. The selected paths are shown in Fig. 27(a). Figure 27(b) shows on-chip loss of paths at 1545 nm excluding loss of fiber-to-chip coupling. The resultant loss was 15.8 dB including 2.1 dB propagation loss of the access waveguides for the input and output, and 13.7 dB loss of the switch part. The propagation loss of the switch part is estimated to be 5.5 dB. The total power consumption for 32 bar-state and 992 cross-state MZ element switches for the full connections was estimated to be 2.93 W. The worst crosstalk was also estimated to be −20 dB at a bandwidth of 1.8 nm. Finally, 32 × 32 optical switch was successfully demonstrated with a 43 Gb/s digital-coherent quadrature phase-shift keying (QPSK) signal at 1544.93 nm wavelength. The current challenge of this work includes taking full advantages of the 32 × 32 switch with the LGA interposer by developing the printed circuit board (PCB) capable of controlling all the 2048 heaters. Broadening the bandwidth, improving the efficiency of optical coupling, and avoiding the input polarization sensitivity are further challenges to enable the practical use of the optical switch.
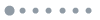
Figure 27.Transmission characteristics of the six sampled optical paths 1-12, 1-20, 1-32, 32-1, 32-13, and 32-19.
(a) Path on the PILOSS switch matrix. (b) On-chip loss. Inset in (b) shows the spectral passband of the path 32-1. Figure reprinted with permission from ref.84, Optical Society of America.
EO based multi-port MZI photonic switch
Si has a large TO coefficient (1.8 × 10−4 K−1) and is used for its TO effect for μs-scale switching87. For ns-scale switching, on the other hand, the plasma dispersion induced EO effects by carrier injection or depletion are widely used because Si has no linear EO effects (Pockels effect). For the fast EO phase shifting, carrier-injection based PIN junctions are more widely applied than their carrier-depletion type PN junction counterparts. However, the EO MZI configuration causes electro-absorption-induced power intensity imbalance in the two parallel arms, thus deteriorating both crosstalk and insertion loss. In 2016, 16 × 16 port-count MZI based Si EO switches were reported88-89. Lu et al. integrated a pair of TiN micro-heaters in both arms and a PIN diode in one arm to optimize device performance88. However, the introduction of a single EO phase shifter only in one arm induced loss and crosstalk imbalances between the cross and bar states due to the free carrier absorption. Qiao et al. implemented PIN junctions in both arms but in an opposite set for push-pull switching operation for mitigating the imbalance of power intensity in two arms89.
Very recently, connectivity of Si EO MZI based switch has been scaled to 32 × 32 by Qiao et al., as shown in Fig. 2890. The switch adopted the Beneš topology using 144 MZI elements. An optical phase bias of π/2 was intentionally introduced to one phase-shifter arm to equalize the phase change of the two arms during push-pull operation. Therefore, operation for the bar and cross states could be balanced and thus optimized. On-chip insertion losses and crosstalk ratios, all in the cross configurations, were measured to be 12.9 ~ 16.5 dB and −17.9 ~ −24.8 dB, respectively, while they were 14.4 ~ 18.5 dB and −15.1 ~ −19.0 dB, all in the bar configurations, respectively. Uniformity of the switch units can be improved by using a more precise process such as 90 ~ 45 nm CMOS technology. Nevertheless, a monitoring method is still needed since several-nm variation in the waveguide width might cause a phase change of π/2 in a 200 μm-long phase shifter. Further optimization is achieved by adding TO heaters to provide an additional control method that succeeds in optimization of both TO and EO phase elements for correcting phase-error and power-imbalance simultaneously91.
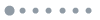
Figure 28.32 × 32 Si EO MZI-based Beneš switch. Figure reprinted with permission from ref.90, under a Creative Commons Attribution 4.0 International License.
Current performances evaluated on loss and crosstalk in these EO switch circuits are not enough for real applications from the practical point of view66. Improvement of such performances in single MZI switch cells has been carried out so far by several methods: introduction of optical phase bias and push-pull control90, application of broadband coupler92 and nested MZI structure93. Some of them succeeded in excellent crosstalk suppression, although insertion loss is more challenging to manage for large-scale switch fabrics. Semiconductor optical amplifiers (SOAs) are reasonable solutions to provide on-chip gain. The recent report on the lossless SOA-integrated 4 × 4 PILOSS Si switch using a flip-chip bonding technique was a notable demonstration94. For the future studies, built-in amplifiers would be needed to build larger Si EO switches for reducing the insertion loss. Polarization-insensitive Si optical switch with the introduction of polarization control elements and polarization-independent Si waveguides would also be another challenge for practical optical communication66.
Multi-port MRR photonic switch
Early research work on MRR based switching circuits was conducted jointly by the teams in Columbia and Cornell, USA95-97. A representative of the hitless router is shown in ref.90, 95. The wavelength-selective nature of the MRR unit requires wavelength alignment across the switching circuit, adding extra overhead. Various schemes for fast and efficient wavelength locking have been demonstrated98-100. The largest port-count of the crossbar type of switch matrix reported to date is 8 × 7 based thermally tuned fifth-order (five-ring series-coupled) Si MRRs90, 101. Single MRR switch element was designed to give a 100 GHz passband and an FSR of 350 GHz. The MRR switch element had an average through-loss of 0.9 dB and drop loss of 2.0 dB. Recently, a modular S & S topology by assembling 1 × N/N × 1 ring-based spatial (de-) multiplexers with low loss fibers or 2D optical interposer were proposed90, 102. An early-stage demonstration of an 8 × 8 S & S MRR switch was performed with excellent results102.
Si photonic multi-port switches are now innovative key devices in the data center as well as in the telecom network. A wide range of Si photonic switches were overviewed in terms of both TO/EO switching effects and MZI/MRR device architectures. Current performance parameters including port count, switching time, power consumption, and optical power penalty are far from being able to implemented in real applications at the moment. Nevertheless, considering the ever-growing interconnect demand of the data center and also remarkable intrinsic feature of the Si photonic platform, several promising answers to the challenges based on the large-scale SOI CMOS integration technologies will be provided in the near future.
Si photonics phased array for LiDAR
In recent years, continuously growing attention toward Si photonic technologies is also extending to unique applications such as light beam manipulating (steering and shaping) in the free space. The currently remarkable challenge in light detection and ranging (LiDAR) is also one of them103. LiDAR is a surveying method for measuring distance to a target by illuminating the target with laser light and detecting the reflected light with a sensor. Differences in laser return times and wavelengths can then be used to make digital 3D representations of the target. LiDAR provides images at higher resolution than that is possible with radar, and has terrestrial, airborne, mobile applications and even applications in point-to-point free-space communication links. In this section, an optical phased array (OPA) developed by Doylend et al is introduced first as an essential element for LiDAR104. Then its application to the LiDAR is reviewed from the first demonstration by Poulton et al105.
OPA
An OPA can be used to steer a beam in free space without mechanical motion106, and has been demonstrated for LiDAR and free-space communication links107, 108. Previous bulk optical components needed to be assembled, aligned and co-packaged. However, new integrated optical components offer several advantages such as small size, free of optical alignment, high tolerance against mechanical vibration and low packaging cost. OPAs can also be realized using Si photonic technologies.
In a standard OPA for 2D beam steering, emitter elements or antennae are arranged in a 2D array and a phase is adjusted to shape/steer the beam. Here, integrated waveguides with surface gratings for free-space emission have the advantage of functioning as 1D phased arrays. Each grating tooth scatters light with a phase delay determined by the effective index of the optical mode. Emission angle is determined by the grating pitch, waveguide effective index and wavelength. For example, a schematic configuration of hybrid Si free-space source with integrated beam steering is shown in Fig. 29(a). Figure 29(b) shows its layout of the overall device demonstrated by Doylend et al104. Here a tunable laser is followed by an SOA pre-amplifier, after which the beam is split into multiple channels (1 × 8 in this case). Each channel is separately amplified and phase tuned before routed to an array of surface gratings. Emission angle of the beam from the surface grating is determined by the wavelength (here symbolized by color) in the θ (longitudinal) axis and by the relative phase in the Ψ axis. They demonstrated a Si 16-channel waveguide surface grating OPA for 2D beam steering. Resultant total field of view, beam width and full-window background peak suppression were 20° × 14°, 0.6° × 1.6° and 10 dB, respectively. Figure 30(a) shows measured beam profiles in the ψ axis for alignment at 1° increments across the field of view, while Fig. 30(b) shows the 3D plots of the beam measured at the corners and center of the field of view109. Afterwards, the Si free-space beam steering chip was first fully integrated using the hybrid Si platform by the same group (Hulme et al)110. Demonstrated photonic integrated circuit (PIC) consists of 164 optical components including lasers, amplifiers, photodiodes, phase tuners, grating couplers, splitters, and a photonic crystal lens. The PIC exhibited steering over 23° × 3.6° with beam widths of 1° × 0.6°.
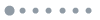
Figure 29.(a) Schematic configuration of hybrid Si free-space source with integrated beam steering. (b) Layout of the overall devices. Figure reprinted with permission from ref.104, SPIE.
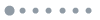
Figure 30.(a) Measured beam profiles at 1555 nm wavelength as the beam was swept across the field of view in the ψ axis at 1° increments. (b) Plots of the 2D beam profiles at the corners and center of the field of view. Figure reprinted with permission from ref.109, Optical Society of America.
LiDAR
LiDAR has been used extensively in autonomous vehicles111, robotics112, aerial mapping113, and atmospheric measurements114. Autonomous systems are key markets for LiDAR devices and, in order to map surrounding environment, solid-state beam steering systems115 have recently been emphasized to increase system durability as compared with the conventional mechanical system such as gimbal. The solid state system has advantages of high scan rate, high reliability and low system cost. In particular, integrated photonics provides a path for low-cost chip-scale LiDAR systems.
In 2017, coherent LiDAR with integrated optical phased arrays for solid-state beam steering was first demonstrated using frequency-modulated continuous-wave (FMCW) LiDAR with a compact laser diode105. FMCW LiDAR allows for simultaneous Doppler-based velocity measurements at no additional cost or complexity. The concept of the FMCW LiDAR is described in detail in ref.116, 117. According to this technique, triangular modulation is used to increase the resolution of the distance/velocity ambiguity caused by the Doppler shift induced from a moving target. Using coherent detection, a time delay between a received frequency-modulated optical signal and a local oscillator (LO) produces an electrical beat frequency. For a stationary target, a single beat frequency proportional to the target distance is observed, while for a moving target, two distinct beat frequencies occur due to the Doppler shift on the received signal. These two beat frequencies occur in different time regions depending on the direction of the laser frequency sweep. Therefore, the difference of the two beat frequencies is twice the induced Doppler shift including the velocity of the target, while the average of the two beat frequencies is proportional to the time-of-flight distance to the target. In this way, by detecting both beat frequencies, distance and velocity can be measured simultaneously. This is the essence of the FMCW LiDAR system.
Figure 31(a) shows a schematic of the solid-state LiDAR system with transmitting (Tx) and receiving (Rx) optical phased arrays (OPAs). In the figure, all the components Tx OPA, Rx OPA and LO are monolithically integrated on the Si chip. Figure 31(b) shows a 3D picture of the optical phased array designed for a solid-state LiDAR system, while Figs. 31(c) to 31(e) show SEM photographs of the fabricated phase shifter, magnified one and antenna. Figure 31(f) shows an optical micrograph of the on-chip Si LiDAR system. The total LiDAR chip let was 6 mm×0.5 mm. Here, the tap from the input waveguide to realize the LO was achieved with a 10% directional coupler. The system was controlled using nine copper electrical pads: three for the Tx array, three for the Rx array, two for the signal and bias of the balanced photodetector, and one for ground. The chip let was packaged in a chip-on-board configuration with an epoxied fiber. The phased array consists of 50 grating-based antennae placed at a 2 μm pitch. The antenna emits a main beam with full-width at half maximum (FWHM) intensity spot size of 0.80° in the array dimension. The antenna length is 500 μm and is designed to have a uniform emission pattern by apodized periodic perturbations on the side of a silicon waveguide. A grouped cascaded phase shifter method was used to apply phase shifts to antenna elements. The light to each antenna is evanescently coupled from a common bus waveguide. The cascaded evanescent couplers have a constant 120 nm coupling gap and coupling lengths that increase by 1.89 μm to create a uniform amplitude distribution. A waveguide embedded thermal phase shifter is placed between each coupler. For beam steering applications, thermal tuning steers in the array dimension, ϕ, while wavelength tuning primarily steers in the antenna dimension, θ. A total steering range of 46° × 36° was achieved with thermal steering under electrical power consumption up to 1.2 W and wavelength tuning from 1454 to 1641 nm.
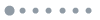
Figure 31.(a) Schematic of the solid-state LiDAR system with transmitting and receiving optical phased arrays. (b) 3D picture of the optical phased array. (c–e) SEM images of phase shifter, magnified one and antenna, respectively. (f) Optical micrograph of the device. Figure reprinted with permission from ref.117, Optical Society of America.
For evaluating the steered LIDAR operation of the device, three targets were placed at different incident angles and different distances, 0.23, 0.32 and 0.45 m apart from the chip. By steering the transmitting and receiving phased arrays simultaneously, each of the targets was measured separately. Although the maximum range and target diffusivity were limited by the aperture size of the phased array performed here, the demonstration conducted here paves the way for on-chip LiDAR systems based on optical phased arrays.
Si photonics for further innovative applications
In Fingertip-size Si photonic interconnect for ultra-short optical links Sections, Si photonic interconnect transceivers and multi-port switches in the telecom/data-com networks, and OPA in LiDAR system have been reviewed as currently attractive applications. Now, novel next-generation applications of Si photonics have been proposed: Ultra-compact optical transceivers for IoTs (internet-of-things) and 5G (fifth generation) networks have already been demonstrated38, 118, while deep learning with coherent nano-photonic circuits119 and neuromorphic photonic integrated circuits120 based on the Si photonics have been intensively discussed in related community. For more details, refer to each reference indicated above.
Recently a new concept of sub-wavelength grating (SWG) constituted of a periodic waveguide with sub-wavelength pitch nanostructure has attracted much attention for its peculiar anisotropic and dispersive behaviors like meta-materials121. It provides possibilities for novel devices such as highly efficient fiber-to-chip couplers, extremely compact beam splitter, polarization management and spectral filtering, contra-directional couplers, etc., all of which are essential building blocks for advanced integrated optical systems. Along with further innovations in process and material technologies as well as device architectures discussed in this paper, silicon photonics will be a promising solution to meet the energy efficiency, sensitivity and cost requirements even in these new applications.
Summary: challenges and perspectives
Si photonics has remarkably progressed owing to its specific features, small footprint, low cost, high energy efficiency and scalability based on the CMOS-process compatibility. They contributed to implementation of a wide range of heterogeneous passive/active optical elements. Among them, indirect-bandgap induced intrinsic difficulty in Si nano-laser has been considerably overcome by Ⅲ-Ⅴ/Si hybrid lasers with chip-/wafer-bonding and Ⅲ-Ⅴ hetero-epitaxial direct growth on the Ge/Si substrate. Nevertheless, considering more advanced integration of these components, further innovative technologies will be required. A through-Si-via (TSV) technology capable of a Si photonic interposer allows for integration of high-performance electronic cores like FPGA together with the driving electronics for the photonics. For material integration for diversification of Si-photonics, Ge-based photonics is also a promising technique allowing for SiGe quantum-well based modulators and detectors.
In application worlds, Si photonic interconnect transducers have advanced far urged by the explosively expanding network traffic in data-com markets. A monolithically integrated "Zero-change" Si photonic transmitter was developed in a 0.03 mm2-sized die by 45 nm SOI CMOS processes and showed 40 Gb/s NRZ transmission characteristics. A fingertip-size optical I/O core with high bandwidth density, low power consumption and high temperature durability was also developed using a 300 mm SOI wafer process and demonstrated 300 m transmission at 25 Gb/s per channel, mounted on the FPGA board. The results are adaptable in the current data-com market.
Si photonics has also been applied to a high-speed and multi-port optical switch with low loss, low cross-talk and polarization-insensitivity in the CDC ROADM telecom network. A multi-cast Si photonic TPA switch with a TO modulator, operating at ~10 μs speed, showed 25 dB average optical loss and 0.15 ms switching time in a 8 × 48 TPA subsystem. The results are useful for future faster ROADM networks. In the data-com network also, dynamic optical path switching using several kinds of multi-port switch topologies have been demonstrated. Ultra-compact 32 × 32 strictly non-blocking Si PILOSS optical switch fabricated using 45 nm CMOS process on the 300 mm wafer demonstrated switching with 43 Gb/s digital-coherent QPSK signal at 1544.93 nm wavelength. Challenges include broader bandwidth, improved optical coupling efficiency and polarization-insensitivity. The switching speed of Si photonics, relying on the TO modulator for μs-scale speed, and EO modulators for ns-scale switching, has been investigated. The EO device depends on plasma effects induced by carrier injection or depletion. Reduction of transmission loss due to the electronic absorption and crosstalk is a main challenge for future demands at ~ns EO switching.
Si photonics applications are extending to novel next-generation systems: optical phased arrays in LiDAR for autonomous systems, ultra-compact optical transceivers in the IoT and 5G networks, deep learning with coherent nano-photonic circuits, neuromorphic photonic integrated circuits, etc. These applications require more advanced Si photonic integration technologies based on new principles and architectures as well as CMOS-compatible processes. Recent novel Si photonic technologies will definitely contribute to the implementation of innovative next-generation Si photonic applications with the support of the intrinsic excellent optical properties of the Si materials.
Acknowledgements
The authors wish to express sincere thanks to Dr. Takahiro Nakamura of PETRA, Dr. Kazuhiko Kurata of AIO Core and Dr. Keizo Kinoshita of AIO Core for helpful discussions on Si photonics technologies and applications.
Competing interests
The authors declare no competing financial interests.
References
[1] Asahi Newspaper article (in Japanese), Jan 9, 2017.
[2] https://www.ntt.co.jp/mirai/e/history/.
[3] R Soref, B Bennett. Electrooptical effects in silicon. IEEE J Quant Electron, 23, 123-129(1987).
[4] G Gunn. CMOS Photonics for high-speed interconnects. IEEE Micro, 26, 58-66(2006).
[5] B Jalali, S Fathpour. Silicon photonics. J Light Wave Technol, 24, 4600-4615(2006).
[6] Z Fang, C Z Zhao. Recent progress in silicon photonics: a review. ISRN Opt, 2012, 428690(2012).
[7] A Dhiman. Silicon photonics: a review. IOSR J Appl Phys (IOSR-JAP), 3, 67-79(2013).
[8] A Kumar. Silicon photonics: An evolving technology. Int J Eng Sci Res Technol, 5, 153-161(2016).
[9] D Thomson, A Zilkie, J E Bowers, T Komljenovic, G T Reed et al. Roadmap on silicon photonics. J Opt, 18, 073003(2016).
[10] J E Bowers, T Komljenovic, M Davenport, J Hulme, A Y Liu et al. Recent advances in silicon photonic integrated circuits. Proc SPIE, 9774, 977402(2017).
[11] V Stojanović, R J Ram, M Popović, S Lin, S Moazeni et al. Monolithic silicon-photonic platforms in state-of-the-art CMOS SOI processes[Invited]. Opt Express, 26, 13106-13121(2018).
[12] Y Hibino. Silica-based planar lightwave circuits and their applications. MRS Bull, 28, 365-371(2003).
[13] G L Bona, R Germann, B J Offrein. SiON high-refractive-index waveguide and planar lightwave circuits. IBM J Res Develop, 47, 239-249(2003).
[14] Y A Vlasov, S J McNab. Losses in single-mode silicon-on-insulator strip waveguides and bends. Opt Express, 12, 1622-1631(2004).
[15] A W Fang, H Park, R Jones, O Cohen, M J Paniccia et al. A continuous-wave hybrid AlGaInAs-silicon evanescent laser. IEEE Photon Technol Lett, 18, 1143-1145(2006).
[16] D Pasquariello, K Hjort. Plasma-assisted InP-to-Si low temperature wafer bonding. IEEE J Sel Top Quant Electron, 8, 118-131(2002).
[17] A Lee, Q Jiang, M C Tang, A Seeds, H Y Liu. Continuous-wave InAs/GaAs quantum-dot laser diodes monolithically grown on Si substrate with low threshold current densities. Opt Express, 20, 22181-22187(2012).
[18] A Y Liu, C Zhang, J Norman, A Snyder, D Lubyshev et al. High performance continuous wave 1.3 μm quantum dot lasers on silicon. Appl Phys Lett, 104, 041104(2014).
[19] H Park, A W Fang, S Kodama, J E Bowers. Hybrid silicon evanescent laser fabricated with a silicon waveguide and Ⅲ-V off set quantum wells. Opt Express, 13, 9460-9464(2005).
[20] G Roelkens, D Van Thourhout, R Baets, R Nötzel, M Smit. Laser emission and photodetection in an InP/InGaAsP layer integrated on and coupled to a silicon-on-insulator waveguide circuit. Opt Express, 14, 8154-8159(2006).
[21] A W Fang, H Park, O Cohen, R Jones, M J Paniccia et al. Electrically pumped hybrid AlGaInAs-silicon evanescent laser. Opt Express, 14, 9203-9210(2006).
[22] D Liang, J E Bowers. Recent progress in lasers on silicon. Nat Photon, 4, 511-517(2010).
[23] M W Maeda, C Chang-Hasnain, A Von Lehmen, H Izadpanah, C Lin et al. Multigigabit/s operations of 16-wavelength vertical-cavity surface-emitting laser array. IEEE Photon Technol Lett, 3, 863-865(1991).
[24] C J Chang-Hasnain. Tunable VCSEL. IEEE J Sel Top Quantum Electron, 6, 978-987(2000).
[25] E Kapon, A Sirbu. Long-wavelength VCSELs: Power-efficient answer. Nat Photon, 3, 27-29(2009).
[26] L Zhu, V Karagodsky, C J Chang-Hasnain. Novel high efficiency vertical to in-plane optical coupler. Proc SPIE, 8270, 82700L(2012).
[27] J Ferrara, W J Yang, L Zhu, P F Qiao, C J Chang-Hasnain. Heterogeneously integrated long-wavelength VCSEL using silicon high contrast grating on an SOI substrate. Opt Express, 23, 2512-2523(2015).
[28] T Akatsu, C Deguet, L Sanchez, F Allibert, D Rouchon et al. Germanium-on-insulator (GeOI) substrates-A novel engineered substrate for future high performance devices. Mater Sci Semicond Proc, 9, 444-448(2006).
[29] H Tanoto, S F Yoon, K L Lew, W K Loke, C Dohrman et al. Electroluminescence and structural characteristics of InAs/In0.9As quantum dots grown on graded Si1-xGex/Si substrate. Appl Phys Lett, 95, 141905(2009).
[30] H Y Liu, T Wang, Q Jiang, R Hogg, F Tutu et al. Long-wavelength InAs/GaAs quantum-dot laser diode monolithically grown on Ge substrate. Nat Photon, 5, 416-419(2011).
[31] A Lee, Q Jiang, M C Tang, A Seeds, H Y Liu. Continuous-wave InAs/GaAs quantum-dot laser diodes monolithically grown on Si substrate with low threshold current densities. Opt Express, 20, 22181-22187(2012).
[32] Seimetz M. Laser linewidth limitations for optical systems with high-order modulation employing feed forward digital carrier phase estimation. In Proceedings of Optical Fiber Communication Conference/National Fiber Optic Engineers Conference OTuM2 (OSA, 2008).
[33] T Komljenovic, S Srinivasan, E Norberg, M Davenport, G Fish et al. Widely tunable narrow-linewidth monolithically integrated external-cavity semiconductor lasers. IEEE J Sel Top Quant Electron, 21, 1501909(2015).
[34] M J R Heck, J F Bauters, , J K Doylend, S Jain et al. Hybird silicon photonic integrated circuit technology. IEEE J Sel Top Quant Electron, 19, 6100117(2013).
[35] F N Xia, L Sekaric, Y Vlasov. Ultracompact optical buffers on a silicon chip. Nat Photon, 1, 65-71(2007).
[36] S Srinivasan, M Davenport, T Komljenovic, J Hulme, D T Spencer et al. Coupled-ring-resonator-mirror-based heterogeneous Ⅲ-V silicon tunable laser. IEEE Photon J, 7, 2700908(2015).
[37] E J Stanton, M J R Heck, J Bovington, A Spott, J E Bowers. Multi-octave spectral beam combiner on ultra-broadband photonic integrated circuit platform. Opt Express, 23, 11272-11283(2015).
[38] H Yaegashi. Development of ultra-compact optical transceivers for IoT network utilizing silicon photonics technology. OKI Tech Rev, 84, 1-4(2017).
[39] B E Little, S T Chu, W Pan, Y Kokubun. Microring resonator arrays for VLSI photonics. IEEE Photon Technol Lett, 12, 323-325(2000).
[40] Q F Xu, D Fattal, R G Beausoleil. Silicon microring resonators with 1. Opt Express, 6, 4309-4315(2008).
[41] https://s3.i-micronews.com/uploads/2020/04/YDR20088-Silicon-Photonics-Market-Technology-2020-Sample.pdf
[42] https://www.eetimes.com/globalfoundries-cuts-5-of-workforce/#. Original source by IC Insights
[43] http://download.intel.com/pressroom/pdf/photonics/50G_Silicon_Photonics_Link.pdf.
[44] R A Soref, J P Lorenzo. Single-crystal silicon: a new material for 1.3 and 1.6 njm integrated-optical components. Electron Lett, 21, 953-954(1985).
[45] G T Reed, W R Headley, C E J Png. Silicon photonics: the early years. Proc SPIE, 5730, 596921(2005).
[46] A Rickman. The commercialization of silicon photonics. Nat Photon, 8, 579-582(2014).
[47] J F Liu, D D Cannon, K Wada, Y Ishikawa, S Jongthammanurak et al. Tensile strained Ge p-i-n photodetectors on Si platform for C and L band telecommunications. Appl Phys Lett, 87, 011110(2005).
[48] 2014 Optical Fiber Communications Conference (OFC) TH4C.1 (OSA, 2014); https://doi.org/10.1364/OFC.2014.Th4C.1.
[49] 2015 Optical Fiber Communications Conference (OFC) W3A.1 (OSA, 2015); https://doi.org/10.1364/OFC.2015.W3A.1.
[50] 2014 Optical Fiber Communications Conference (OFC) Th5C.1 (OSA, 2014); https://doi.org/10.1364/OFC.2014.Th5C.1.
[51] Silicon photonics shipments, for datacenter (In units) 2019-2025e. Yole Développement (2020).
[52] Patterson D, De Sousa I, Achard L M. The future of packaging with silicon photonics. Chip Scale Rev, 1-10 (Jan, 2017).
[53] https://arstechnica.com/information-technology/2015/05/ibm-demos-first-fully-integrated-monolithic-silicon-photonics-chip/.
[54] S. Narasimha, K. Onishi, H. M. Nayfeh, A. Waite, M. Weybright et al. High performance 45-nm SOI technology with enhanced strain, porous Low-k BEOL, and immersion lithography. In Proceedings of International Electron Devices Meeting, (IEEE, 2006), 1-4.
[55] J S Orcutt, B Moss, C Sun, J Leu, M Georgas et al. Open foundry platform for high-performance electronic-photonic integration. Opt Express, 20, 12222-12232(2012).
[56] C Sun, M Wade, M Georgas, S Lin, L Alloatti et al. A 45 nm CMOS-SOI Monolithic photonics platform with bit-statistics-based resonant microring thermal tuning. IEEE J Solid-State Circuits, 51, 893-907(2016).
[57] ESSCIRC 2014-40th European Solid State Circuits Conference (ESSCIRC) 199-202 (IEEE, 2014); http://doi.org/10.1109/ESSCIRC.2014.6942056.
[58] 2017 IEEE 25th Symposium on High-Performance Interconnects (HOTI) 25-28 (IEEE, 2017); http://doi.org/10.1109/HOTI.2017.23.
[59] S Moazeni, S Lin, M T Wade, L Alloatti, R J Ram et al. A 40Gb/s PAM-4 transmitter based on a ring-resonator optical DAC in 45nm SOI CMOS. IEEE J Solid-State Circuits, 52, 3503-3516(2017).
[60] T Nakamura, K Yashiki, K Mizutani, T Nedachi, J Fujikata et al. Fingertip-size optical module, pOptical I/O Coreq, and its application in FPGA. IEICE Trans Electron, E102-C, 333-339(2019).
[61] http://www.aiocore.com/technology
[62] T Mogami, T Horikawa, K Kinoshita, Y Hagihara, J Ushida et al. 1.2 Tbps/cm2 enabling silicon photonics IC technology based on 40-nm generation platform. J Lightw Technol, 36, 4701-4712(2018).
[63] 2015 IEEE 65th Electronic Components and Technology Conference (ECTC) (IEEE, 2015); http://doi.org/10.1109/ECTC.2015.7159766.
[64] Yashiki K, Mizutani K, Ushida J, Suzuki Y, Kurihara M et al. 25-Gbps error-free operation of chip-scale Si-photonics optical transmitter over 70℃ with integrated quantum dot laser. In Optical Fiber Communications Conference and Exhibition (OFC) Th1F.7 (OSA, 2016).
[65] https://www.corning.com/media/worldwide/coc/documents/Fiber/PI1468_07-14_English.pdf.
[66] Q X Cheng, M Bahadori, M Glick, S Rumley, K Bergman. Recent advances in optical technologies for data centers: a review. Optica, 5, 1354-1370(2018).
[67] H S Hinton. An Introduction to Photonic Switching Fabrics(2013).
[68] 2016 21st OptoElectronics and Communications Conference (OECC) held jointly with 2016 International Conference on Photonics in Switching (PS) (IEEE, 2016). https://ieeexplore.ieee.org/document/7718222.
[69] Y Sakamaki, T Kawai, M Fukutoku. Next-generation optical switch technologies for realizing ROADM with more flexible functions. NTT Tech Rev, 12, 1-5(2014).
[70] E B Basch, R Egorov, S Gringeri, S Elby. Architectural tradeoffs for reconfigurable dense wavelength-division multiplexing systems. IEEE J Sel Top Quant Electron, 12, 615-626(2006).
[71] S Gringeri, B Basch, V Shukla, R Egorov, T J Xia. Flexible architectures for optical transport nodes and networks. IEEE Commun Mag, 48, 40-50(2010).
[72] https://www.cisco.com/c/dam/m/en_us/network-intelligence/service-provider/digital-transformation/knowledge-network-webinars/pdfs/1213-business-services-ckn.pdf.
[73] H Takeshita, T Hino, K Ishii, J Kurumida. Prototype highly integrated 8×48 transponder aggregator based on Si photonics for multi-degree colorless, directionless, contentionless reconfigurable optical add/drop multiplexer. IEICE Trans Electron, E96-C, 966-973(2013).
[74] E Yamazaki, S Yamanaka, Y Kisaka, T Nakagawa, K Murata et al. Fast optical channel recovery in field demonstration of 100-Gbit/s Ethernet over OTN using real-time DSP. Opt Express, 19, 13179-13184(2011).
[75] S Nakamura, S Yanagimachi, H Takeshita, A Tajima, T Hino et al. Optical switches based on silicon photonics for ROADM application. IEEE J Sel Top Quant Electron, 22, 3600609(2016).
[76] Optical Fiber Communication Conference OTu2I.3 (OSA, 2012); https://doi.org/10.1364/OFC.2012.OTu2I.3.
[77] Optical Fiber Communication Conference M2B.6 (OSA, 2015); https://doi.org/10.1364/OFC.2015.M2B.6.
[78] Asia Communications and Photonics Conference AF4B.3 (OSA, 2014); https://doi.org/10.1364/ACPC.2014.AF4B.3.
[79] G Cocorullo, Corte F G Della, I Rendina. Temperature dependence of the thermo-optic coefficient in crystalline silicon between room temperature and. Appl Phys Lett, 74, 3338-3340(1999).
[80] 2014 the European Conference on Optical Fiber Communications (ECOC 2014) PD.1.3 (IEEE, 2014); http://doi.org/10.1109/ECOC.2014.6964268.
[81] Optical Fiber Communication Conference OTuM2 (OSA, 2011). https://doi.org/10.1364/OFC.2011.OTuM2.
[82] L Chen, Y K Chen. Compact, low-loss and low-power 8×8 broadband silicon optical switch. Opt Express, 20, 18977-18985(2012).
[83] K Suzuki, K Tanizawa, T Matsukawa, G W Cong, S H Kim et al. Ultra-compact 8×8 strictly-non-blocking Si-wire PILOSS switch. Opt Express, 22, 3887-3894(2014).
[84] K Tanizawa, K Suzuki, M Toyama, M Ohtsuka, N Yokoyama et al. Ultra-compact 32×32 strictly-non-blocking Si-wire optical switch with fan-out LGA interposer. Opt Express, 23, 17599-17606(2015).
[85] T Goh, A Himeno, M Okuno, H Takahashi, K Hattori. High-extinction ratio and low-loss silica-based 8×8 strictly nonblocking thermooptic matrix switch. J Lightwave Technol, 17, 1192-1199(1999).
[86] 2006 European Conference on Optical Communication (ECOC 2006) OThV4 (IEEE, 2006); http://doi.org/10.1109/ECOC.2006.4801113.
[87] M Bahadori, A Gazman, N Janosik, S Rumley, Z Y Zhu et al. Thermal rectification of integrated microheaters for microring resonators in silicon photonics platform. J Lightwave Technol, 36, 773-788(2017).
[88] L J Lu, S Y Zhao, L J Zhou, D Li, Z X Li et al. 16×16 non-blocking silicon optical switch based on electro-optic Mach-Zehnder interferometers. Opt Express, 24, 9295-9307(2016).
[89] 2016 Optical Fiber Communications Conference and Exhibition (OFC), Anaheim, CA, 1-3 (IEEE, 2016). https://ieeexplore.ieee.org/document/7537295.
[90] L Qiao, W J Tang, T Chu. 32×32 silicon electro-optic switch with built-in monitors and balanced-status units. Sci Rep, 7, 42306(2017).
[91] Optical Fiber Communication Conference Th1G.2 (OSA, 2018); http://doi.org/10.1364%2FOFC.2018.Th1G.2.
[92] N Dupuis, B G Lee, A V Rylyakov, D M Kuchta, C W Baks et al. Design and fabrication of low-insertion-loss and low-crosstalk broadband 2×2 Mach-zehnder silicon photonic switches. J Lightwave Technol, 33, 3597-3606(2015).
[93] N Dupuis, A V Rylyakov, C L Schow, D M Kuchta, C W Baks et al. Ultralow crosstalk nanosecond-scale nested 2×2 Mach-Zehnder silicon photonic switch. Opt Lett, 41, 3002-3005(2016).
[94] Optical Fiber Communication Conference Th4B.6 (OSA, 2018); http://doi.org/10.1364/OFC.2018.Th4B.6.
[95] N Sherwood-Droz, H Wang, L Chen, B G Lee, A Biberman et al. Optical 4×4 hitless silicon router for optical networks-on-chip (NoC). Opt Express, 16, 15915-15922(2008).
[96] B G Lee, A Biberman, P Dong, M Lipson, K Bergman. All-optical comb switch for multiwavelength message routing in silicon photonic networks. IEEE Photonics Technol Lett, 20, 767-769(2008).
[97] A Biberman, H L R Lira, K Padmaraju, N Ophir, J Chan et al. Broadband silicon photonic electrooptic switch for photonic interconnection networks. IEEE Photonics Technol Lett, 23, 504-506(2011).
[98] A S P Khope, T Hirokawa, A M Netherton, M Saeidi, Y Xia et al. On-chip wavelength locking for photonic switches. Opt Lett, 42, 4934-4937(2017).
[99] K Padmaraju, D F Logan, T Shiraishi, J J Ackert, A P Knights et al. Wavelength locking and thermally stabilizing microring resonators using dithering signals. J Lightwave Technol, 32, 505-512(2014).
[100] X L Zhu, K Padmaraju, L W Luo, S Yang, M Glick et al. Fast wavelength locking of a microring resonator. IEEE Photonics Technol Lett, 26, 2365-2368(2014).
[101] P DasMahapatra, R Stabile, A Rohit, K A Williams. Optical crosspoint matrix using broadband resonant switches. IEEE J Sel Top Quant Electron, 20, 5900410(2014).
[102] D Nikolova, D M Calhoun, Y Liu, S Rumley, A Novack et al. Modular architecture for fully non-blocking silicon photonic switch fabric. Microsyst Nanoeng, 3, 16071(2017).
[103] R M Marino, Jr W R Davis. Jigsaw: a foliage-penetrating 3D imaging laser radar system. Lincoln Lab J, 15, 23-36(2005).
[104] J K Doylend, M J R Heck, J T Bovington, J D Peters, M L Davenport et al. Hybrid silicon free-space source with integrated beam steering. Proc SPIE, 8629, 862911(2013).
[105] C V Poulton, A Yaacobi, D B Cole, M J Byrd, M Raval et al. Coherent solid-state LIDAR with silicon photonic optical phased arrays. Opt Lett, 42, 4091-4094(2017).
[106] P F McManamon, P J Bos, M K Escuti, J Heikenfeld, S Serati et al. A review of phased array steering for narrow-band electrooptical systems. Proc IEEE, 97, 1078-1096(2009).
[107] A Schweinsberg, Z M Shi, J E Vornehm, R W Boyd. A slow-light laser radar system with two-dimensional scanning. Opt Lett, 37, 329-331(2012).
[108] C J Henderson, D G Leyva, T D Wilkinson. Free space adaptive optical interconnect at 1.25 Gb/s, with beam steering using a ferroelectric liquid-crystal SLM. J Lightwave Technol, 24, 1989-1997(2006).
[109] J K Doylend, M J R Heck, J T Bovington, J D Peters, L A Coldren et al. Two-dimensional free-space beam steering with an optical phased array on silicon-on-insulator. Opt Express, 19, 21595-21604(2011).
[110] J C Hulme, J K Doylend, M J R Heck, J D Peters, M L Davenport et al. Fully integrated hybrid silicon two dimensional beam scanner. Opt Express, 23, 5861-5874(2015).
[111] 2011 IEEE Intelligent Vehicles Symposium (IV) 163-168 (IEEE, 2011); http://doi.org/10.1109/IVS.2011.5940562.
[112] J F Lalonde, N Vandapel, D F Huber, M Hebert. Natural terrain classification using three-dimensional ladar data for ground robot mobility. J Field Robot, 23, 839-861(2006).
[113] Y J Lin, J Hyyppä, A Jaakkola. Mini-UAV-borne LIDAR for fine-scale mapping. IEEE Geosci Remote Sens Lett, 8, 426-430(2011).
[114] J H Schween, A Hirsikko, U Löhnert, S Crewell. Mixing-layer height retrieval with ceilometer and Doppler lidar: from case studies to long-term assessment. Atmos Meas Tech, 7, 3685-3704(2014).
[115] S R Davis, S D Rommel, D Gann, B Luey, J D Gamble et al. A lightweight, rugged, solid state laser radar system enabled by non-mechanical electro-optic beam steerers. Proc SPIE, 9832, 98320K(2016).
[116] Proceedings of the Materials Research Society Symposium, San Francisco, CA, USA, 1076 (2008); http://doi.org/10.1557/PROC-1076-K04-06.
[117] C V Poulton, A Yaacobi, D B Cole, M J Byrd, M Raval et al. Large-scale silicon nitride nanophotonic phased arrays at infrared and visible wavelengths. Opt Lett, 42, 21-24(2017).
[118] P Iovanna, F Cavaliere, F Testa, S Stracca, G Botarri et al. Future proof optical network infrastructure for 5G transport. J Opt Commun Netw, 8(2016).
[119] Y C Shen, N C Harris, S Skirlo, M Prabhu, T B Jones et al. Deep learning with coherent nanophotonic circuits. Nat Photon, 11, 441-446(2017).
[120] H T Peng, M A Nahmias, T F de Lima, A N Tait, B J Shastri. Neuromorphic photonic integrated circuits. IEEE J Sel Top Quant Electron, 24, 6101715(2018).
[121] P Cheben, R Halir, J H Schmid, H A Atwater, D R Smith. Subwavelength integrated photonics. Nature, 560, 565-572(2018).