Xinwei Li, Dasom Kim, Yincheng Liu, Junichiro Kono, "Terahertz spin dynamics in rare-earth orthoferrites," Photon. Insights 1, R05 (2022)

Search by keywords or author
- Photonics Insights
- Vol. 1, Issue 2, R05 (2022)
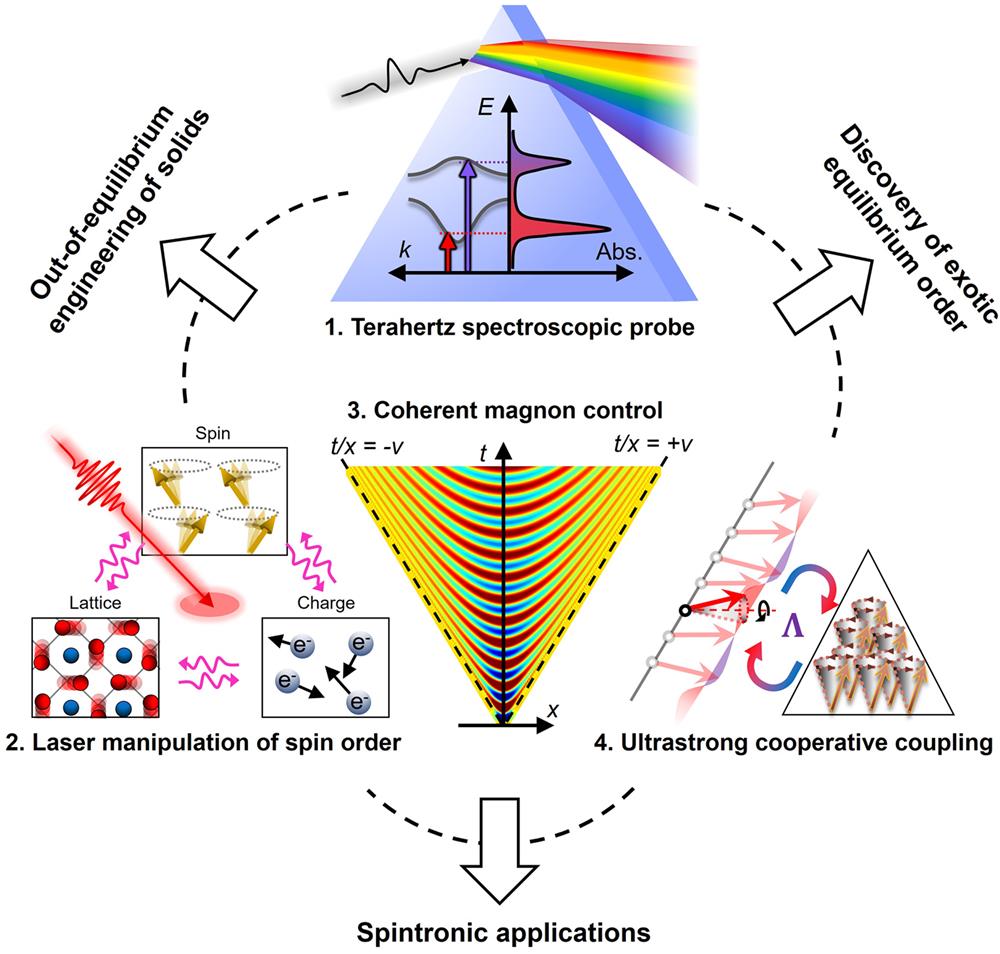
Fig. 1. Overview of current scientific and technological interests related to spin dynamics in solid-state materials. Four topics arranged as smaller triangular elements are covered in this review, which are key elements for achieving the three grander goals (three sides of the larger triangle).
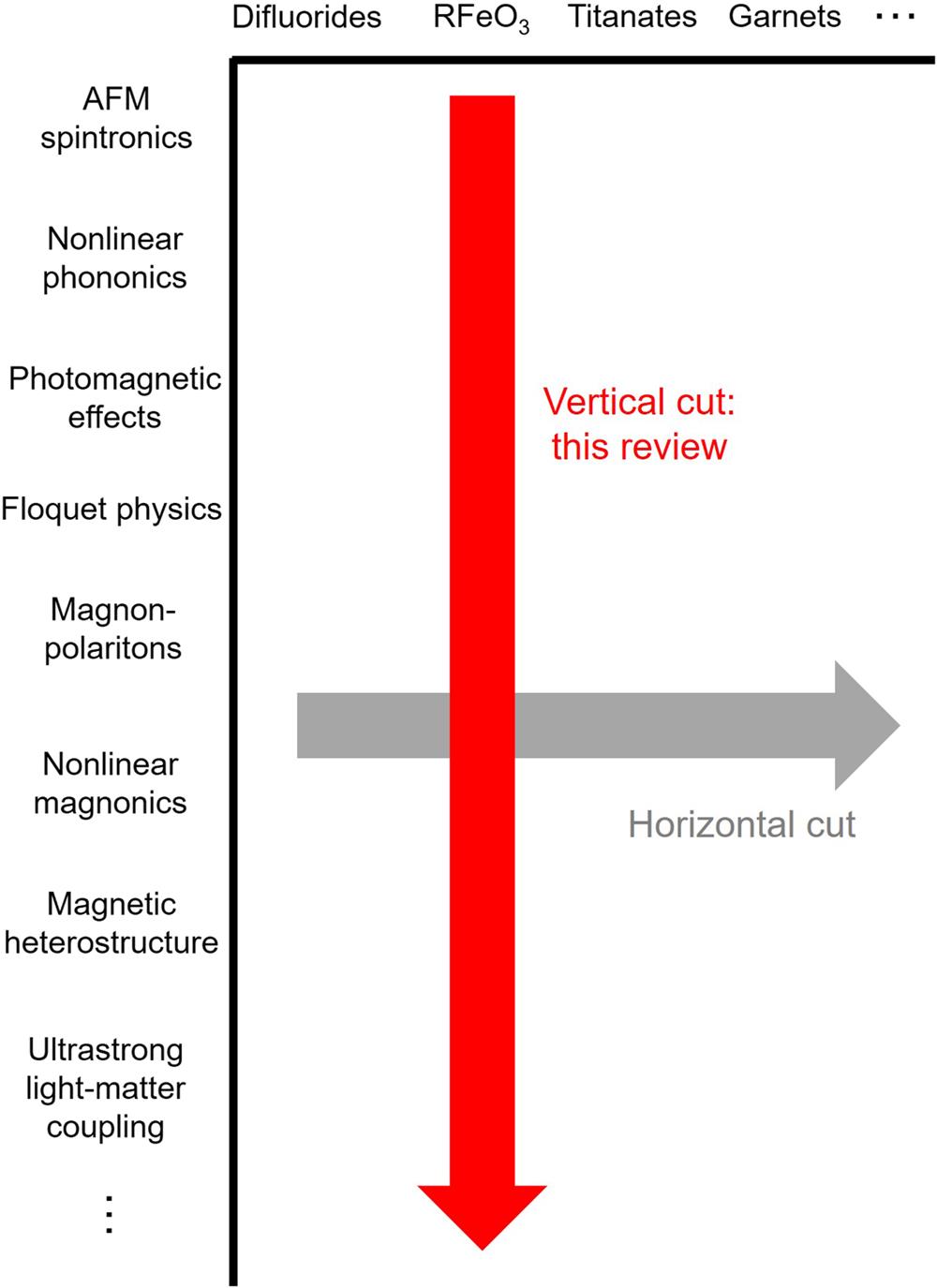
Fig. 2. “Phase space” for review articles in spintronics, spanned by the horizontal axis of materials and vertical axis of novel physical phenomena. The current review represents a vertical cut in the phase space.
![Temperature-dependent magnetic phase diagrams for all members of the RFeO3 class[79], except for R=Pm, for which no data are available due to its radioactivity. SRT, spin reorientation transition. Reproduced with permission from Ref. [79].](/Images/icon/loading.gif)
Fig. 3. Temperature-dependent magnetic phase diagrams for all members of the class[79], except for , for which no data are available due to its radioactivity. SRT, spin reorientation transition. Reproduced with permission from Ref. [79].
![Basic physical properties of RFeO3 crystals. (a) Temperature-dependent magnetization of LuFeO3, ErFeO3, and DyFeO3 along the z axis and x axis[75]. (b) Optical absorption spectrum of ErFeO3[94]. (c) Spin-wave dispersion mapped by inelastic neutron scattering[95]. (d) Spin oscillations in quasi-FM and quasi-AFM modes[96]. Each spin evolves in the sequence of 1→2→3→4→1…. Spins are synchronized by the number label. Reproduced with permission from Refs. [75,94–96].](/Images/icon/loading.gif)
Fig. 4. Basic physical properties of crystals. (a) Temperature-dependent magnetization of , , and along the axis and axis[75]. (b) Optical absorption spectrum of [94]. (c) Spin-wave dispersion mapped by inelastic neutron scattering[95]. (d) Spin oscillations in quasi-FM and quasi-AFM modes[96]. Each spin evolves in the sequence of . Spins are synchronized by the number label. Reproduced with permission from Refs. [75,94– 96].
![Observation of (a) the inverse Faraday effect[118] and (b) the inverse Cotton–Mouton effect[119] in DyFeO3. The oscillation phases of magnons excited by these photomagnetic effects depend on the polarization of the pump light. (c) Magnon excitation by the two photomagnetic effects can be described by the framework of impulsive stimulated Raman scattering[57]. Reproduced with permission from Refs. [57,118,119].](/Images/icon/loading.gif)
Fig. 5. Observation of (a) the inverse Faraday effect[118] and (b) the inverse Cotton–Mouton effect[119] in . The oscillation phases of magnons excited by these photomagnetic effects depend on the polarization of the pump light. (c) Magnon excitation by the two photomagnetic effects can be described by the framework of impulsive stimulated Raman scattering[57]. Reproduced with permission from Refs. [57,118,119].
![Path of solving for the full dynamics of photomagnetic pump, magneto-optical probe experiments on RFeO3, summarized from the procedure adopted in Ref. [119].](/Images/icon/loading.gif)
Fig. 6. Path of solving for the full dynamics of photomagnetic pump, magneto-optical probe experiments on , summarized from the procedure adopted in Ref. [119].
![THz time-domain techniques. (a) Layout of a THz time-domain spectroscopy setup configured in a transmission geometry. (b) Zoom-in view of the polarization-sensitive differential detection setup[36]. (c) Layout of a THz emission spectroscopy setup. (d) Function of a reflective echelon used in a single-shot THz spectrometer[138]. (e) Pulse-front-tilt technique for generating intense THz radiation in LiNbO3[38]. BS, beam splitter; QWP, quarter-wave plate; WP, Wollaston prism; BD, balanced detector. (b), (d), (e) Reproduced with permission from Refs. [36,38,138].](/Images/icon/loading.gif)
Fig. 7. THz time-domain techniques. (a) Layout of a THz time-domain spectroscopy setup configured in a transmission geometry. (b) Zoom-in view of the polarization-sensitive differential detection setup[36]. (c) Layout of a THz emission spectroscopy setup. (d) Function of a reflective echelon used in a single-shot THz spectrometer[138]. (e) Pulse-front-tilt technique for generating intense THz radiation in [38]. BS, beam splitter; QWP, quarter-wave plate; WP, Wollaston prism; BD, balanced detector. (b), (d), (e) Reproduced with permission from Refs. [36,38,138].
![Six measurement configurations, along with the magnon polarization selection rule in the Γ4 and Γ2 phases. The magnetic field component of the THz pulse HTHz is polarized along a line whose color indicates the magnon mode that can be excited. The THz propagation direction is perpendicular to the disk containing the line. Reproduced with permission from Ref. [169].](/Images/icon/loading.gif)
Fig. 8. Six measurement configurations, along with the magnon polarization selection rule in the and phases. The magnetic field component of the THz pulse is polarized along a line whose color indicates the magnon mode that can be excited. The THz propagation direction is perpendicular to the disk containing the line. Reproduced with permission from Ref. [169].
![Temperature-dependent SRT probed by THz spectroscopy[170–172" target="_self" style="display: inline;">–172]. (a) Switching of the polarization selection rule. (b) Switching of the polarization trajectory of magnon emission. (c) Experimental verification of (b). (d) Continuous spectral weight transfer between two measurement configurations of the quasi-AFM mode amplitude. (e), (f) Quantifying the rotation angle during the SRT from quasi-AFM to quasi-FM mode spectral weight transfer in z-cut Dy0.7Er0.3FeO3. Inset to (f): calculated free-energy landscape at various temperatures (red to blue: low to high temperatures). Reproduced with permission from Refs. [170–172" target="_self" style="display: inline;">–172].](/Images/icon/loading.gif)
Fig. 9. Temperature-dependent SRT probed by THz spectroscopy[170– 172" target="_self" style="display: inline;">– 172 ]. (a) Switching of the polarization selection rule. (b) Switching of the polarization trajectory of magnon emission. (c) Experimental verification of (b). (d) Continuous spectral weight transfer between two measurement configurations of the quasi-AFM mode amplitude. (e), (f) Quantifying the rotation angle during the SRT from quasi-AFM to quasi-FM mode spectral weight transfer in -cut . Inset to (f): calculated free-energy landscape at various temperatures (red to blue: low to high temperatures). Reproduced with permission from Refs. [170– 172" target="_self" style="display: inline;">– 172 ].
![Magnetic-field-induced Γ4→Γ2 SRT probed by THz spectroscopy[174,175]. (a) Observation of switching of the polarization selection rule in NdFeO3. (b) Field-dependent absorption spectra in YFeO3. (c) Calculated quasi-FM and quasi-AFM frequencies versus magnetic field, to compare with (b). Reproduced with permission from Refs. [174,175].](/Images/icon/loading.gif)
Fig. 10. Magnetic-field-induced SRT probed by THz spectroscopy[174,175]. (a) Observation of switching of the polarization selection rule in . (b) Field-dependent absorption spectra in . (c) Calculated quasi-FM and quasi-AFM frequencies versus magnetic field, to compare with (b). Reproduced with permission from Refs. [174,175].
![CFTs of Tm3+ ions in TmFeO3[179]. (a) Absorption coefficient mapped as a function of frequency and temperature. Red circles: quasi-AFM mode. Blue squares: quasi-FM mode. (b) Constant-temperature cut of absorption coefficient at 60 K for three different measurement configurations. (c) Energy-level diagram explaining the THz transitions. Reproduced with permission from Ref. [179].](/Images/icon/loading.gif)
Fig. 11. CFTs of ions in [179]. (a) Absorption coefficient mapped as a function of frequency and temperature. Red circles: quasi-AFM mode. Blue squares: quasi-FM mode. (b) Constant-temperature cut of absorption coefficient at 60 K for three different measurement configurations. (c) Energy-level diagram explaining the THz transitions. Reproduced with permission from Ref. [179].
![CFTs of Er3+ in ErFeO3[169]. (a) Energy-level scheme of Er3+. Levels shown in red lines are involved in the transitions observed in experiments. (b) Two-sublattice model. Magneto-THz absorption spectra from measurements in (c) configuration 1, (d) configuration 4, and (e) configuration 6. (f)–(h) Energy-level calculations from the best fit of experimental spectra using the two-sublattice model, corresponding to the configurations in (c)–(e), respectively. Reproduced with permission from Ref. [169].](/Images/icon/loading.gif)
Fig. 12. CFTs of in [169]. (a) Energy-level scheme of . Levels shown in red lines are involved in the transitions observed in experiments. (b) Two-sublattice model. Magneto-THz absorption spectra from measurements in (c) configuration 1, (d) configuration 4, and (e) configuration 6. (f)–(h) Energy-level calculations from the best fit of experimental spectra using the two-sublattice model, corresponding to the configurations in (c)–(e), respectively. Reproduced with permission from Ref. [169].
![Studying Er3+ CFTs in ErFeO3 by THz emission spectroscopy[180]. (a) Pump-helicity-dependent THz emission from y-cut ErFeO3. (b) Fourier transform of the helicity-dependent contribution in (a). (c) Mode symmetry assignments determined from the polarization of emission and pump-helicity dependence. Measurement configuration is the same as (a) and (b). Gray region indicates the SRT temperature range. Crystal-field states are labeled with the same notations as in Fig. 12(a). Reproduced with permission from Ref. [180].](/Images/icon/loading.gif)
Fig. 13. Studying CFTs in by THz emission spectroscopy[180]. (a) Pump-helicity-dependent THz emission from -cut . (b) Fourier transform of the helicity-dependent contribution in (a). (c) Mode symmetry assignments determined from the polarization of emission and pump-helicity dependence. Measurement configuration is the same as (a) and (b). Gray region indicates the SRT temperature range. Crystal-field states are labeled with the same notations as in Fig. 12(a) . Reproduced with permission from Ref. [180].
![Electromagnons in DyFeO3 and TbFeO3[195,196] measured by far-infrared transmittance spectroscopy. (a) Zero-field mode excitations as a function of temperature in the ETHz∥z, HTHz∥y geometry for DyFeO3. (b) Magnetic field dependence of DyFeO3 transmittance using the ETHz∥z, HTHz∥x geometry for B∥y at 1.5 K. Up and down sweeps are shown in the left and right panels, respectively. (c) Zero-field temperature-dependent scans using the ETHz∥z, HTHz∥x geometry for TbFeO3. Right panel zooms in the left panel. (d) Magnetic field dependence of TbFeO3 transmittance for B∥y at 1.5 K. Reproduced with permission from Refs. [195,196].](/Images/icon/loading.gif)
Fig. 14. Electromagnons in and [195,196] measured by far-infrared transmittance spectroscopy. (a) Zero-field mode excitations as a function of temperature in the , geometry for . (b) Magnetic field dependence of transmittance using the , geometry for at 1.5 K. Up and down sweeps are shown in the left and right panels, respectively. (c) Zero-field temperature-dependent scans using the , geometry for . Right panel zooms in the left panel. (d) Magnetic field dependence of transmittance for at 1.5 K. Reproduced with permission from Refs. [195,196].
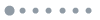
Fig. 15. Three-temperature model. (a) Separate but mutually interacting reservoirs. (b) Time dynamics of temperatures of the reservoirs.
![Temperature dependence of the anisotropy constants, Ax and Az, in TmFeO3[179]. Reproduced with permission from Ref. [179].](/Images/icon/loading.gif)
Fig. 16. Temperature dependence of the anisotropy constants, and , in [179]. Reproduced with permission from Ref. [179].
![Ultrafast-heating-induced SRT in TmFeO3[225]. (a) Equilibrium linear birefringence as a function of temperature. (b) Time-resolved pump–probe linear birefringence traces at different temperatures. (c) Temperature dependence of the amplitudes of magnon oscillations and reorientation amplitude extracted by fitting to the data in (b). Reproduced with permission from Ref. [225].](/Images/icon/loading.gif)
Fig. 17. Ultrafast-heating-induced SRT in [225]. (a) Equilibrium linear birefringence as a function of temperature. (b) Time-resolved pump–probe linear birefringence traces at different temperatures. (c) Temperature dependence of the amplitudes of magnon oscillations and reorientation amplitude extracted by fitting to the data in (b). Reproduced with permission from Ref. [225].
![(a) Ultrafast-heating-induced SRT in ErFeO3 probed by time-resolved Faraday rotation[226]. (b) THz pump Faraday rotation probe experiment on ErFeO3[227]. Quasi-FM mode whose frequency is stable with time is observed. (c) Same experiment as (b) except that an additional near-infrared pulse excites the sample at 20 ps to produce ultrafast heating. The temperature was 30 K. (d) Time evolution of the anisotropy energy due to ultrafast heating extracted by fitting the magnon frequency shift in (c). (e) Energy-transfer scheme among three reservoirs during ultrafast heating[226]. 3d electrons and phonon quickly thermalize upon excitation, but the energy transfer rate between 4f ions and lattice depends on the 4f ion species. The model resembles the three-temperature model in essence, but slightly differs in the definition of reservoirs. Reproduced with permission from Refs. [226,227].](/Images/icon/loading.gif)
Fig. 18. (a) Ultrafast-heating-induced SRT in probed by time-resolved Faraday rotation[226]. (b) THz pump Faraday rotation probe experiment on [227]. Quasi-FM mode whose frequency is stable with time is observed. (c) Same experiment as (b) except that an additional near-infrared pulse excites the sample at 20 ps to produce ultrafast heating. The temperature was 30 K. (d) Time evolution of the anisotropy energy due to ultrafast heating extracted by fitting the magnon frequency shift in (c). (e) Energy-transfer scheme among three reservoirs during ultrafast heating[226]. electrons and phonon quickly thermalize upon excitation, but the energy transfer rate between ions and lattice depends on the ion species. The model resembles the three-temperature model in essence, but slightly differs in the definition of reservoirs. Reproduced with permission from Refs. [226,227].
![Reconfigurable magnetic domains in ErFeO3 by static heating from a THz free-electron laser (FEL)[229]. (a) Sequence of Faraday rotation microscopy images showing spin-up domain expansion when the FEL spot (macropulse energy: 8 mJ) scans on the sample surface. Red circle: FEL focal spot. (b) Active area of domain flipping is off from the center of the laser spot. Reproduced with permission from Ref. [229].](/Images/icon/loading.gif)
Fig. 19. Reconfigurable magnetic domains in by static heating from a THz free-electron laser (FEL)[229]. (a) Sequence of Faraday rotation microscopy images showing spin-up domain expansion when the FEL spot (macropulse energy: 8 mJ) scans on the sample surface. Red circle: FEL focal spot. (b) Active area of domain flipping is off from the center of the laser spot. Reproduced with permission from Ref. [229].
![Comparison of time scales between (a) photomagnetic-effect-induced SRT and (b) laser-heating-induced SRT, analyzed by extracting the polarization-dependent and polarization-independent probe responses, respectively[226]. Reproduced with permission from Ref. [226].](/Images/icon/loading.gif)
Fig. 20. Comparison of time scales between (a) photomagnetic-effect-induced SRT and (b) laser-heating-induced SRT, analyzed by extracting the polarization-dependent and polarization-independent probe responses, respectively[226]. Reproduced with permission from Ref. [226].
![Inertia-driven SRT in HoFeO3[231]. (a) Intense magnetic field pulse generated by IFE supplies enough kinetic energy to enable spin switching to a metastable state. In HoFeO3, the two free-energy minima coincide with the Γ12 and Γ24 phases, distinguished by an azimuthal angle ϕ of the AFM vector. (b) Observation of spin switching when the photomagnetic pulse exceeds a critical field strength. (c) Time scale of IFE-induced switching is much faster than the heating time scale. Reproduced with permission from Ref. [231].](/Images/icon/loading.gif)
Fig. 21. Inertia-driven SRT in [231]. (a) Intense magnetic field pulse generated by IFE supplies enough kinetic energy to enable spin switching to a metastable state. In , the two free-energy minima coincide with the and phases, distinguished by an azimuthal angle of the AFM vector. (b) Observation of spin switching when the photomagnetic pulse exceeds a critical field strength. (c) Time scale of IFE-induced switching is much faster than the heating time scale. Reproduced with permission from Ref. [231].
![Domain-controllable laser-induced SRT due to the combined effect of IFE and ultrafast heating[232]. (a) Faraday rotation images taken after a single shot of pump pulse by various delay times around the Γ2→Γ4 SRT temperature range. Gray corresponds to the Γ2 phase. Black (white) corresponds to a spin-down (-up) domain in the Γ4 phase. (b) Mechanism for controllable switching. Reproduced with permission from Ref. [232].](/Images/icon/loading.gif)
Fig. 22. Domain-controllable laser-induced SRT due to the combined effect of IFE and ultrafast heating[232]. (a) Faraday rotation images taken after a single shot of pump pulse by various delay times around the SRT temperature range. Gray corresponds to the phase. Black (white) corresponds to a spin-down (-up) domain in the phase. (b) Mechanism for controllable switching. Reproduced with permission from Ref. [232].
![Domain-controllable SRT in ErFeO3 using THz-NIR double pumping[233]. (a) THz pump field (enhanced by a metamaterial) first launches a coherent magnon, and depending on the timing of the NIR heating pulse within the magnon oscillation period, the SRT can be controlled to show a single domain. The sample temperature is 84 K. (b) 2D plot of Faraday rotation as a function of probe time delay t and THz-NIR time interval dt. (c) Final-state magnetization as a function of dt has opposite phase when probing inside and outside the metamaterial ring, suggesting the SRT pathway is sensitive to the sign of the THz magnetic field. Reproduced with permission from Ref. [233].](/Images/icon/loading.gif)
Fig. 23. Domain-controllable SRT in using THz-NIR double pumping[233]. (a) THz pump field (enhanced by a metamaterial) first launches a coherent magnon, and depending on the timing of the NIR heating pulse within the magnon oscillation period, the SRT can be controlled to show a single domain. The sample temperature is 84 K. (b) 2D plot of Faraday rotation as a function of probe time delay and THz-NIR time interval . (c) Final-state magnetization as a function of has opposite phase when probing inside and outside the metamaterial ring, suggesting the SRT pathway is sensitive to the sign of the THz magnetic field. Reproduced with permission from Ref. [233].
![Solution of QIR and QR from Eq. (18) upon pulsed laser excitation whose center frequency is resonant with ωIR[206].](/Images/icon/loading.gif)
Fig. 24. Solution of and from Eq. (18 ) upon pulsed laser excitation whose center frequency is resonant with [206].
![Nonlinear phononic control of magnetic phases in DyFeO3[238]. (a) Frequency shifts (marked by red and blue arrows) of the quasi-AFM mode due to phonon pumping. (b) Frequency shift as a function of pump photon energy. Shaded curve shows the phonon absorption spectrum. (c) Faraday rotation transients with increasing pump fluence at 45 K. (d) Offset versus fluence. (e) Offset versus temperature for fluences below (red) and above (blue) the threshold. Ic denotes the fluence threshold. (f) Phonon pumping versus charge pumping (ultrafast heating). Reproduced with permission from Ref. [238].](/Images/icon/loading.gif)
Fig. 25. Nonlinear phononic control of magnetic phases in [238]. (a) Frequency shifts (marked by red and blue arrows) of the quasi-AFM mode due to phonon pumping. (b) Frequency shift as a function of pump photon energy. Shaded curve shows the phonon absorption spectrum. (c) Faraday rotation transients with increasing pump fluence at 45 K. (d) Offset versus fluence. (e) Offset versus temperature for fluences below (red) and above (blue) the threshold. denotes the fluence threshold. (f) Phonon pumping versus charge pumping (ultrafast heating). Reproduced with permission from Ref. [238].
![Evolution of the amplitude of the two Raman modes with (a) 45 deg and (b) –45 deg pump polarizations. Notice the sign change of the B1g mode[240]. Insets show shifts of the free-energy minima of the Raman modes. Reproduced with permission from Ref. [240].](/Images/icon/loading.gif)
Fig. 26. Evolution of the amplitude of the two Raman modes with (a) 45 deg and (b) –45 deg pump polarizations. Notice the sign change of the mode[240]. Insets show shifts of the free-energy minima of the Raman modes. Reproduced with permission from Ref. [240].
![Phonon IFE in ErFeO3[241]. (a) Eigenvectors of in-plane IR phonons, and their superposition. (b) Time-resolved Faraday rotation transients under different THz pump polarizations. Oscillations contain fast Raman phonons and the slow quasi-AFM magnon (outlined by thick solid curves). No magnon is observed when the pump polarization aligns with a crystal axis. (c) Quadratic dependence of magnon amplitude on the pump field. Reproduced with permission from Ref. [241].](/Images/icon/loading.gif)
Fig. 27. Phonon IFE in [241]. (a) Eigenvectors of in-plane IR phonons, and their superposition. (b) Time-resolved Faraday rotation transients under different THz pump polarizations. Oscillations contain fast Raman phonons and the slow quasi-AFM magnon (outlined by thick solid curves). No magnon is observed when the pump polarization aligns with a crystal axis. (c) Quadratic dependence of magnon amplitude on the pump field. Reproduced with permission from Ref. [241].
![Nonlinear excitation of magnons by pumping rare-earth crystal-field transitions[244]. (a) Intense THz pump repopulates Tm3+ ions within its crystal-field levels, which in turn modifies the magnetic anisotropy. (b) Faraday rotation transients under increasing THz pump fields. (c) Fourier transform of (b). (d) Spectral weights of the quasi-FM and quasi-AFM modes extracted from (c). (e) Nonlinearity of quasi-FM excitation versus temperature. Reproduced with permission from Ref. [244].](/Images/icon/loading.gif)
Fig. 28. Nonlinear excitation of magnons by pumping rare-earth crystal-field transitions[244]. (a) Intense THz pump repopulates ions within its crystal-field levels, which in turn modifies the magnetic anisotropy. (b) Faraday rotation transients under increasing THz pump fields. (c) Fourier transform of (b). (d) Spectral weights of the quasi-FM and quasi-AFM modes extracted from (c). (e) Nonlinearity of quasi-FM excitation versus temperature. Reproduced with permission from Ref. [244].
![Comparing the onset of magnetic anisotropy due to rare-earth pumping and phonon pumping[245]. (a) Pathway that leads to anisotropy modification. 25 THz pump drives optical phonons, while 33 THz pump drives Sm3+ atomic transitions. Lower: anisotropy energy landscape within the SRT temperature range. Orientation of the easy axis is parameterized by θ. Under laser illumination, the easy axis switches to a new direction of θ+Δθ. (b) Faraday rotation transients at various temperatures within the SRT range for a 25 THz pump and a 33 THz pump. (c) Detailed comparison of the rise time of the offset signal. (d) Rise time for establishing anisotropy for the two types of pumps. Blue: 33 THz pump. Red: 25 THz pump. Inset zooms into the 312–320 K range. Reproduced with permission from Ref. [245].](/Images/icon/loading.gif)
Fig. 29. Comparing the onset of magnetic anisotropy due to rare-earth pumping and phonon pumping[245]. (a) Pathway that leads to anisotropy modification. 25 THz pump drives optical phonons, while 33 THz pump drives atomic transitions. Lower: anisotropy energy landscape within the SRT temperature range. Orientation of the easy axis is parameterized by . Under laser illumination, the easy axis switches to a new direction of . (b) Faraday rotation transients at various temperatures within the SRT range for a 25 THz pump and a 33 THz pump. (c) Detailed comparison of the rise time of the offset signal. (d) Rise time for establishing anisotropy for the two types of pumps. Blue: 33 THz pump. Red: 25 THz pump. Inset zooms into the 312–320 K range. Reproduced with permission from Ref. [245].
![Floquet spectrum and exchange interaction energy of a two-site cluster Hubbard model[256]. (a) Energy-level structure versus the Floquet parameter E. (b) Exchange interaction versus E for two pumping frequencies. The unit is the hopping amplitude t0. Onsite repulsion is assumed to be U=10. The red dashed-dotted line and blue dashed line are obtained with perturbation theory (valid for E≪1). Reproduced with permission from Ref. [256].](/Images/icon/loading.gif)
Fig. 30. Floquet spectrum and exchange interaction energy of a two-site cluster Hubbard model[256]. (a) Energy-level structure versus the Floquet parameter . (b) Exchange interaction versus for two pumping frequencies. The unit is the hopping amplitude . Onsite repulsion is assumed to be . The red dashed-dotted line and blue dashed line are obtained with perturbation theory (valid for ). Reproduced with permission from Ref. [256].
![Floquet modification of exchange interaction in RFeO3[123]. (a) Infrared pump pulses (centered at 1.55 eV) nonresonantly drive a Fe-O-Fe cluster. (b) Ultrafast modification of exchange interaction is due to virtual charge transfer arising from light-induced orbital state mixing. (c) Temperature-dependent THz emission signal for ErFeO3. Reproduced with permission from Ref. [123].](/Images/icon/loading.gif)
Fig. 31. Floquet modification of exchange interaction in [123]. (a) Infrared pump pulses (centered at 1.55 eV) nonresonantly drive a Fe-O-Fe cluster. (b) Ultrafast modification of exchange interaction is due to virtual charge transfer arising from light-induced orbital state mixing. (c) Temperature-dependent THz emission signal for . Reproduced with permission from Ref. [123].
![High Q factors of THz magnons in RFeO3. (a) Table summarized using data from Ref. [140], which lists frequency f, linewidth Δf (in FWHM), and Q factor for quasi-FM and quasi-AFM magnons in various RFeO3 crystals at room temperature. (b) Long-lived temporal oscillations due to quasi-FM magnons in ErFeO3 at 20 K[275]. The bottom row shows zoom-in views of −4–21 ps, 500–535 ps, and 2005–2040 ps. Reproduced with permission from Ref. [275].](/Images/icon/loading.gif)
Fig. 32. High factors of THz magnons in . (a) Table summarized using data from Ref. [140], which lists frequency , linewidth (in FWHM), and factor for quasi-FM and quasi-AFM magnons in various crystals at room temperature. (b) Long-lived temporal oscillations due to quasi-FM magnons in at 20 K[275]. The bottom row shows zoom-in views of −4–21 ps, 500–535 ps, and 2005–2040 ps. Reproduced with permission from Ref. [275].
![Double-pulse coherent control of magnons in YFeO3[284]. (a) Mach–Zender-type interferometer setup used to achieve NOT and XNOR gates[7,283]. (b) Double-pulse pumping experiment on an x-cut crystal. Left: time-domain signals with single-pulse and double-pulse excitations. Right: Fourier transform of the FID signal. (c) Coherent control of the quasi-FM mode in a z-cut crystal. THz electric field is parallel along the y axis. (d) Fourier transform of the FID signal in (c) versus inter-pulse time delay. (e) Energy lost in the magnon sector due to destructive interference is restored back to the second pulse. Reproduced with permission from Refs. [7,284].](/Images/icon/loading.gif)
Fig. 33. Double-pulse coherent control of magnons in [284]. (a) Mach–Zender-type interferometer setup used to achieve NOT and XNOR gates[7,283]. (b) Double-pulse pumping experiment on an -cut crystal. Left: time-domain signals with single-pulse and double-pulse excitations. Right: Fourier transform of the FID signal. (c) Coherent control of the quasi-FM mode in a -cut crystal. THz electric field is parallel along the axis. (d) Fourier transform of the FID signal in (c) versus inter-pulse time delay. (e) Energy lost in the magnon sector due to destructive interference is restored back to the second pulse. Reproduced with permission from Refs. [7,284].
![Single-pulse coherent control of magnons in YFeO3[285]. (a) Polarization configuration. (b) FID signal versus the polarization angle θ. (c) FID signal amplitude (derived from Fourier transform, shown in red circles) versus θ. Black solid line is model calculation using Eq. (31). Blue dashed and green dotted lines consider either the birefringence or the dichroism (but not both). Reproduced with permission from Ref. [285].](/Images/icon/loading.gif)
Fig. 34. Single-pulse coherent control of magnons in [285]. (a) Polarization configuration. (b) FID signal versus the polarization angle . (c) FID signal amplitude (derived from Fourier transform, shown in red circles) versus . Black solid line is model calculation using Eq. (31 ). Blue dashed and green dotted lines consider either the birefringence or the dichroism (but not both). Reproduced with permission from Ref. [285].
![Magnon–phonon-polaritons in a photonic crystal cavity[295]. (a) Experimental configuration. (b) Electro-optic sampling imaging of the cavity mode 3 ps after pump excitation. (c) Anticrossing branches of the magnon–phonon-polariton. Temperature is adjusted to detune the magnon and the phonon-polariton frequencies. Gray and yellow markers: data. Solid lines: polariton branches. Dashed lines: mode frequencies assuming no coupling. Reproduced with permission from Ref. [295].](/Images/icon/loading.gif)
Fig. 35. Magnon–phonon-polaritons in a photonic crystal cavity[295]. (a) Experimental configuration. (b) Electro-optic sampling imaging of the cavity mode 3 ps after pump excitation. (c) Anticrossing branches of the magnon–phonon-polariton. Temperature is adjusted to detune the magnon and the phonon-polariton frequencies. Gray and yellow markers: data. Solid lines: polariton branches. Dashed lines: mode frequencies assuming no coupling. Reproduced with permission from Ref. [295].
![Magnon-polaritons in a Fabry–Pérot cavity[296]. (a) Experimental phase shift of ∂S21/∂T. Dashed lines mark the polariton modes. (b) Model simulations. Dashed green lines: uncoupled modes. Modes with strong (weak) temperature dependence are magnon (cavity) modes. Purple solid lines: polariton modes. Reproduced with permission from Ref. [296].](/Images/icon/loading.gif)
Fig. 36. Magnon-polaritons in a Fabry–Pérot cavity[296]. (a) Experimental phase shift of . Dashed lines mark the polariton modes. (b) Model simulations. Dashed green lines: uncoupled modes. Modes with strong (weak) temperature dependence are magnon (cavity) modes. Purple solid lines: polariton modes. Reproduced with permission from Ref. [296].
![Magnon–phonon-polaritons in a hybrid waveguide[295]. (a) Experimental configuration. (b) Dispersion relation of transverse-electric phonon-polariton modes. (c) Zoom-in view of the red-oval-enclosed region, where polariton branches form an anticrossing pattern. Reproduced with permission from Ref. [295].](/Images/icon/loading.gif)
Fig. 37. Magnon–phonon-polaritons in a hybrid waveguide[295]. (a) Experimental configuration. (b) Dispersion relation of transverse-electric phonon-polariton modes. (c) Zoom-in view of the red-oval-enclosed region, where polariton branches form an anticrossing pattern. Reproduced with permission from Ref. [295].
![Signatures of magnon-polaritons in free space[297]. (a) As optically excited magnons propagate in a TmFeO3 crystal, they couple with a free-space light field and form polariton modes. (b) FID signal in transmission experiments (left) and its Fourier transform (right). (c) Emission fields in THz emission experiment (left) and its Fourier transform (right). Simulation results (black lines and gray shades) are overlaid on data (red hollow circles). Temperature: 40 K. Reproduced with permission from Ref. [297].](/Images/icon/loading.gif)
Fig. 38. Signatures of magnon-polaritons in free space[297]. (a) As optically excited magnons propagate in a crystal, they couple with a free-space light field and form polariton modes. (b) FID signal in transmission experiments (left) and its Fourier transform (right). (c) Emission fields in THz emission experiment (left) and its Fourier transform (right). Simulation results (black lines and gray shades) are overlaid on data (red hollow circles). Temperature: 40 K. Reproduced with permission from Ref. [297].
![Demonstration of magnon propagation at a supersonic group velocity[299]. (a) High-k magnons can be excited if pump excitation is confined in a very small penetration depth. Inset: penetration depth and absorption coefficient versus photon energy. (b) Within the Faraday and Kerr measurement geometries, the Kerr geometry is sensitive to high-k modes. (c) Simulated chirping of the magnon wave packet. (d) Reconstruction of the magnon dispersion relation by probe-wavelength-dependent measurements using the Kerr geometry. (e) Group velocity plot obtained from the dispersion. Colored markers: experimental data. Black dashed lines: sound velocity vs and limiting group velocity v0. Reproduced with permission from Ref. [299].](/Images/icon/loading.gif)
Fig. 39. Demonstration of magnon propagation at a supersonic group velocity[299]. (a) High- magnons can be excited if pump excitation is confined in a very small penetration depth. Inset: penetration depth and absorption coefficient versus photon energy. (b) Within the Faraday and Kerr measurement geometries, the Kerr geometry is sensitive to high- modes. (c) Simulated chirping of the magnon wave packet. (d) Reconstruction of the magnon dispersion relation by probe-wavelength-dependent measurements using the Kerr geometry. (e) Group velocity plot obtained from the dispersion. Colored markers: experimental data. Black dashed lines: sound velocity and limiting group velocity . Reproduced with permission from Ref. [299].
![Efficient magnon excitation in Fe/RFeO3 heterostructure[305]. (a) Magnetic hysteresis loops of the Fe/ErFeO3(100) sample for various field orientations. Fields never exceed the coercive field of RFeO3. (b) Time-resolved MOKE transients for Fe/ErFeO3(100) and the bare ErFeO3(100) substrate under comparable pump fluences. (c) Proposed microscopic mechanism that explains efficient magnon excitation across a wide temperature range. Spins are canted initially due to interfacial exchange (left). Quenching of exchange by optical pulses leads to a restoring force and thereby launches magnons (right). Reproduced with permission from Ref. [305].](/Images/icon/loading.gif)
Fig. 40. Efficient magnon excitation in heterostructure[305]. (a) Magnetic hysteresis loops of the sample for various field orientations. Fields never exceed the coercive field of . (b) Time-resolved MOKE transients for and the bare substrate under comparable pump fluences. (c) Proposed microscopic mechanism that explains efficient magnon excitation across a wide temperature range. Spins are canted initially due to interfacial exchange (left). Quenching of exchange by optical pulses leads to a restoring force and thereby launches magnons (right). Reproduced with permission from Ref. [305].
![Correlated SRT in Co/SmFeO3 heterostructure[306,307]. (a) Experimental configuration. (b) Heating-induced SRT in the Co film probed by XMCD imaging. (c) Analogous maps to (b) except that heating is supplied by a laser beam. (d) Time-resolved XMCD images before and after a pulsed optical pump, and the pump-induced change obtained by a subtraction. Reproduced with permission from Refs. [306,307].](/Images/icon/loading.gif)
Fig. 41. Correlated SRT in heterostructure[306,307]. (a) Experimental configuration. (b) Heating-induced SRT in the Co film probed by XMCD imaging. (c) Analogous maps to (b) except that heating is supplied by a laser beam. (d) Time-resolved XMCD images before and after a pulsed optical pump, and the pump-induced change obtained by a subtraction. Reproduced with permission from Refs. [306,307].
![Nonlinearity of magnons as evidence for all-coherent spin switching[312]. (a) Experimental configuration. (b) Spin orientations in the Γ24 phase and the two potential energy minima centered at φ0 and φ1. (c) Spin trajectories with (red curve) and without (blue curve) spin switching. Inset: modification to the potential due to an impulsive THz drive. (d) Fluence dependence of Faraday rotation transients. Dashed line highlights the phase shift. (e) Fourier transform of signals in (d). (f) Fluence dependence of the long-lived offset signal. Gray area marks the fluence range higher than the switching threshold. Reproduced with permission from Ref. [312].](/Images/icon/loading.gif)
Fig. 42. Nonlinearity of magnons as evidence for all-coherent spin switching[312]. (a) Experimental configuration. (b) Spin orientations in the phase and the two potential energy minima centered at and . (c) Spin trajectories with (red curve) and without (blue curve) spin switching. Inset: modification to the potential due to an impulsive THz drive. (d) Fluence dependence of Faraday rotation transients. Dashed line highlights the phase shift. (e) Fourier transform of signals in (d). (f) Fluence dependence of the long-lived offset signal. Gray area marks the fluence range higher than the switching threshold. Reproduced with permission from Ref. [312].
![2D coherent THz spectroscopy[313]. (a) Experimental configuration. (b) Various signal fields with either a single pulse or double pulses. The nonlinear signal BNL(t,τ) is obtained by a subtraction process achieved in the experiment by a differential chopping detection method. (c), (d) 2D magnitude spectra of BAB(f,ν) for measurements focusing on the quasi-AFM and quasi-FM frequency ranges. (e) Double-sided Feynman diagrams showing the excitation pathways of various nonlinear processes marked in (c) and (d). Blue and red arrows represent interactions with pulses A and B, respectively. Black dashed arrow represents the measured emission field. Reproduced with permission from Ref. [313].](/Images/icon/loading.gif)
Fig. 43. 2D coherent THz spectroscopy[313]. (a) Experimental configuration. (b) Various signal fields with either a single pulse or double pulses. The nonlinear signal is obtained by a subtraction process achieved in the experiment by a differential chopping detection method. (c), (d) 2D magnitude spectra of for measurements focusing on the quasi-AFM and quasi-FM frequency ranges. (e) Double-sided Feynman diagrams showing the excitation pathways of various nonlinear processes marked in (c) and (d). Blue and red arrows represent interactions with pulses A and B, respectively. Black dashed arrow represents the measured emission field. Reproduced with permission from Ref. [313].
![Light–matter interaction setup for studying the Dicke phase transition in the USC regime[337]. (a) N atoms in a photonic cavity. (b) Diagram of an individual atom. (c) Static cavity and matter fields (upper)[338] and polariton frequencies versus coupling strength (lower) calculated from the Dicke model. (d) Same as the lower panel of (c), but for the Hopfield model (full Hamiltonian retaining the A2-term). Reproduced with permission from Ref. [337].](/Images/icon/loading.gif)
Fig. 44. Light–matter interaction setup for studying the Dicke phase transition in the USC regime[337]. (a) atoms in a photonic cavity. (b) Diagram of an individual atom. (c) Static cavity and matter fields (upper)[338] and polariton frequencies versus coupling strength (lower) calculated from the Dicke model. (d) Same as the lower panel of (c), but for the Hopfield model (full Hamiltonian retaining the -term). Reproduced with permission from Ref. [337].
![Spin–magnon interaction in ErFeO3[169]. Quasi-FM oscillations of Fe3+ are resonantly coupled with the EPR of NEr3+ spins at a rate of Λ. Reproduced with permission from Ref. [169].](/Images/icon/loading.gif)
Fig. 45. Spin–magnon interaction in [169]. Quasi-FM oscillations of are resonantly coupled with the EPR of spins at a rate of . Reproduced with permission from Ref. [169].
![Evidence for Dicke cooperativity in magnetic interactions[169]. (a)–(k) THz absorption spectra of ErFeO3 crystal under a z-oriented external magnetic field at various temperatures and Y3+ doping levels. (l) The coupling rate shows proportionality with the square root of Er3+ density. Inset shows two types of mechanisms that tune the effective spin density of Er3+. Reproduced with permission from Ref. [169].](/Images/icon/loading.gif)
Fig. 46. Evidence for Dicke cooperativity in magnetic interactions[169]. (a)–(k) THz absorption spectra of crystal under a -oriented external magnetic field at various temperatures and doping levels. (l) The coupling rate shows proportionality with the square root of density. Inset shows two types of mechanisms that tune the effective spin density of . Reproduced with permission from Ref. [169].
![Magnonic superradiant phase transition in ErFeO3[356]. (a), (b) Γ2→Γ12 LTPT, across which Er3+ develops AFM order and Fe3+ spins rotate in the b−c plane. Averaged spin components of (c) Er3+ and (d) Fe3+ versus temperature calculated via the mean-field method. (e) Temperature–field phase diagram for BDC∥x, highlighting the phase boundary of LTPT, calculated by the mean-field method. (f) Phase boundaries calculated from the extended Dicke Hamiltonian, by selectively including certain terms in the Hamiltonian. Solid: both the Er3+–magnon and Er3+−Er3+ interactions are included. Dashed-dotted: only the Er3+−Er3+ interaction is included. Dashed: only the Er3+–magnon interaction is included. Reproduced with permission from Ref. [356].](/Images/icon/loading.gif)
Fig. 47. Magnonic superradiant phase transition in [356]. (a), (b) LTPT, across which develops AFM order and spins rotate in the b −c plane. Averaged spin components of (c) and (d) versus temperature calculated via the mean-field method. (e) Temperature–field phase diagram for , highlighting the phase boundary of LTPT, calculated by the mean-field method. (f) Phase boundaries calculated from the extended Dicke Hamiltonian, by selectively including certain terms in the Hamiltonian. Solid: both the –magnon and interactions are included. Dashed-dotted: only the interaction is included. Dashed: only the –magnon interaction is included. Reproduced with permission from Ref. [356].
![Temperature-field phase diagram for BDC∥z[358]. Between the normal “N” phase and the superradiant “S” phase, a new intermediate “I” phase emerges. The S-to-I transition is first-order. The S-to-N and I-to-N transitions are both second-order.](/Images/icon/loading.gif)
Fig. 48. Temperature-field phase diagram for [358]. Between the normal “N” phase and the superradiant “S” phase, a new intermediate “I” phase emerges. The S-to-I transition is first-order. The S-to-N and I-to-N transitions are both second-order.
![YFeO3 as a quantum simulator of the anisotropic Hopfield Hamiltonian[359]. (a) Experimental geometry. (b) Experimental mode frequencies (with error bars) versus magnetic field for various tilt angles θ. Theoretical mode calculations using the full Hamiltonian (red solid) and decoupled Hamiltonian (black dashed) are overlaid. (c) g1 and g2 versus field for various θ. (d) g1 and g2 versus θ at the zero-detuning magnetic field. Reproduced with permission from Ref. [359].](/Images/icon/loading.gif)
Fig. 49. as a quantum simulator of the anisotropic Hopfield Hamiltonian[359]. (a) Experimental geometry. (b) Experimental mode frequencies (with error bars) versus magnetic field for various tilt angles . Theoretical mode calculations using the full Hamiltonian (red solid) and decoupled Hamiltonian (black dashed) are overlaid. (c) and versus field for various . (d) and versus at the zero-detuning magnetic field. Reproduced with permission from Ref. [359].
![Perfect intrinsic squeezing at an SRPT critical point[338]. Minimized quadrature fluctuation (red) versus coupling strength shows perfect suppression at the SRPT transition point. Upper panel shows complementary polariton mode frequencies.](/Images/icon/loading.gif)
Fig. 50. Perfect intrinsic squeezing at an SRPT critical point[338]. Minimized quadrature fluctuation (red) versus coupling strength shows perfect suppression at the SRPT transition point. Upper panel shows complementary polariton mode frequencies.
|
|
Table 2. Property Tensors, Their Contributions to the Hamiltonian, and Their Resulting Magneto-Optical and Photomagnetic Effects[57]a .
|
Table 3. Crystal-Field Parameters for Levels | m = ± 15 / 2 〉 and | m = ± 13 / 2 〉 Obtained from the Fits to the 80 K Experimental Spectra Shown in Fig. 12 (reproduced with permission from Ref. [169]).
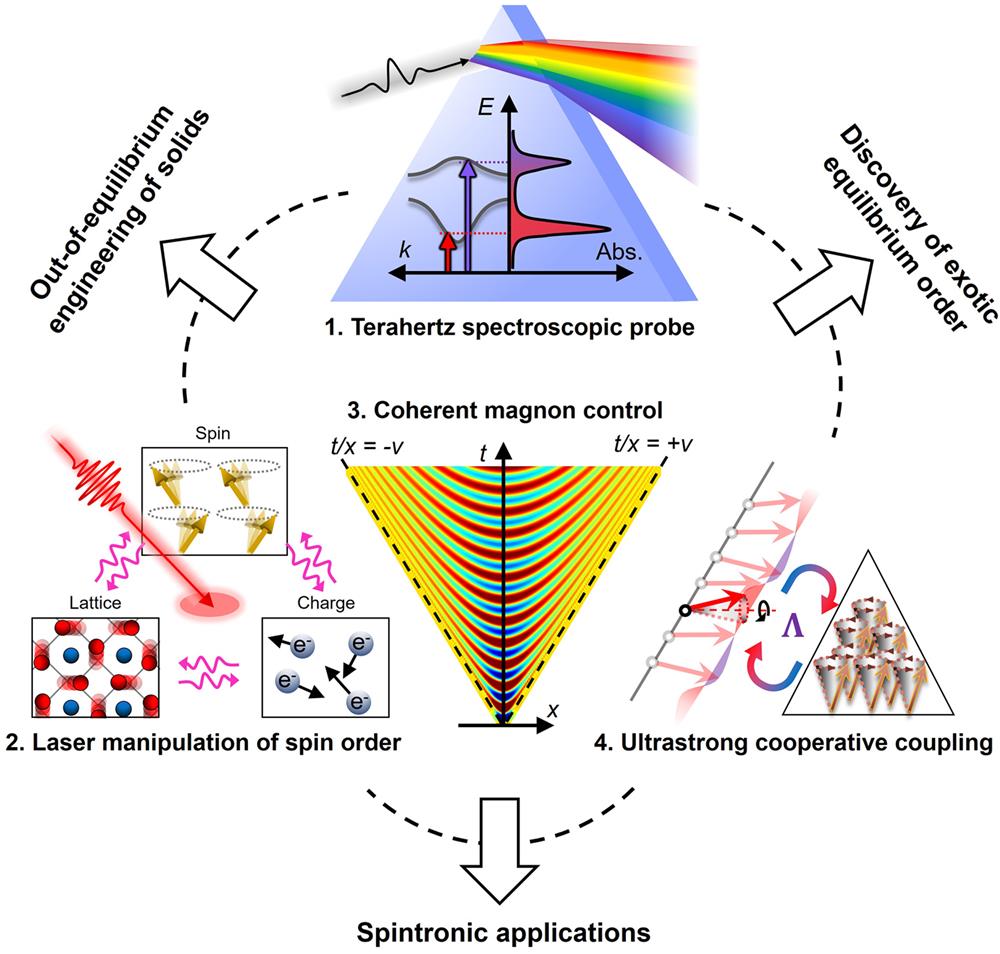
Set citation alerts for the article
Please enter your email address