
- Photonics Research
- Vol. 9, Issue 12, 2420 (2021)
Abstract
1. INTRODUCTION
X-ray has the ability to interact with electrons in the atoms to ionize targeted materials and shows a good dose-dependent feature [1,2]. Therefore, X-ray detection is of great significance for medical imaging, security screening, industrial nondestructive inspection, scientific research, etc. [3–5]. To meet these applications, high detection sensitivity is crucial for achieving high-contrast images and reducing radiation-related health risks [6,7].
Typically, two different methods are adopted for X-ray detection. The dominant indirect-conversion X-ray detectors use phosphors or scintillators to transform an X-ray into visible light so the spatial resolution and conversion efficiency are limited [8,9]. In contrast, the direct-conversion X-ray detectors use a semiconductor layer to convert an X-ray directly into electric signals and show the advantages of high spatial resolution, fast response time, and wide linear dynamic range [10,11]. However, the commercial a-Se photoconductor used in direct-conversion X-ray detectors has the drawback of low X-ray absorption coefficient for general radiology applications, resulting in a limited sensitivity of
In addition, the X-ray detection sensitivity can be tremendously improved using the photoelectron multiplication mechanism. There are three main photoelectron multiplication mechanisms for realizing highly sensitive X-ray detection: photoemission and secondary emission in vacuum photomultiplier tubes (PMTs) [17], avalanche multiplication mechanism in avalanche photodiodes (APDs) and pixelated silicon photomultipliers (SiPMs) [18,19], and photoconductive gain in X-ray detectors with electronic injection at high electric field [20]. However, commercial PMTs have a bulky size and high cost; the APDs and SiPMs have drawbacks of temperature-dependent gain and low X-ray absorption coefficient due to material limitation; the X-ray detectors with photoconductor gain suffer from the issue of a current runaway effect that occurs at a high electric field so the gain is limited. Therefore, it is challenging to develop a flat-panel X-ray detector with simple preparation method, high-stability, high X-ray absorption coefficient, and high internal gain.
Sign up for Photonics Research TOC. Get the latest issue of Photonics Research delivered right to you!Sign up now
In order to develop a flat-panel X-ray detector for highly sensitive and low-dose X-ray detection, vacuum cold cathode flat-panel X-ray detectors have been fabricated successfully in recent years and achieve high internal gain [21,22]. The detectors consist of a-Se high-gain avalanche rushing photoconductors (HARPs) for photoelectric conversion and Spindt-type field emission arrays (FEAs) for signal readout [21]. Those vacuum X-ray detectors achieved a pixel number of
Here, the direct-conversion vacuum cold cathode X-ray detectors consisting of ZnO NW field emitters and
2. RESULTS AND DISCUSSION
Conventional direct-conversion X-ray detectors with thin film transistor (TFT) readout are illustrated in Fig. 1(a) [4]. The EHPs are generated in a photoconductor through the absorption of X-ray photons. Nowadays, a-Se photoconductors used in commercial X-ray detectors have drawbacks of low X-ray absorption efficiency and charge collection efficiency. The developing X-ray detectors based on CdTe [27], PbO [28],
Figure 1.Comparison of conceptual design of X-ray detectors and corresponding X-ray response mechanism. (a) Conventional X-ray detectors with TFT readout. (b) Proposed X-ray detectors with vacuum FEA readout.
The proposed device structure formed by gated FEAs and photoconductor target for achieving low dose X-ray imaging is illustrated in Fig. 1(b). These vacuum cold cathode X-ray detectors with high-resolution imaging (20 μm) and high-radiation tolerance (
Our device adopts a vacuum photodiode formed by a
Figure 2.Implementation and characterizations of the X-ray detectors. (a) Schematic layout of the vacuum cold cathode X-ray detector. (b) Actual picture of the fabricated X-ray detector. (c) Arrays of patterned ZnO NWs (top image) and cross-sectional view of ZnO NWs (bottom image). (d) Field emission
In addition, the charge collection efficiency of the X-ray detector is related to the field emission characteristics of ZnO NWs and the performance metrics of
In order to obtain the crystallinity of
In addition to exploring the charge transport mechanism of the detector to achieve maximum X-ray detection sensitivity, the dark current and photocurrent were measured at low and high electric fields [Figs. 3(a) and 3(b)]. The nonzero dark current and photocurrent at zero electric field shown in Fig. 3(a) might be caused by the trapped electron in the
Figure 3.Detection performance and operation principle of the proposed X-ray detector. (a), (b) Dark current and photocurrent of the X-ray detector at low and high electric fields. (c) F-N curves of the dark current and photocurrent. (d)–(f) Schematic of the operation principle of X-ray detector at different applied electric fields. (g) The detection sensitivity versus applied electric field curves of the X-ray detector. (h) Internal gain versus electric field curve of the detector under X-ray illumination with the X-ray tube voltage of 6 kV and the X-ray tube current of 0.6 mA. (i) The detection sensitivity versus X-ray tube voltage curves of the X-ray detector with inset showing the theoretical determination of detector sensitivity as a function of X-ray energy. (The experimental value was measured using a DC X-ray tube without beam filters.)
The operation principle of the X-ray detector is illustrated to further demonstrate the I-E characteristics and X-ray response process at different electric fields. As shown in Figs. 3(d)–3(f), the charge transport mechanism of the X-ray detector can be divided into three regions. In Region I, the internal photoelectric effect of X-ray photons in the
Under the EBIPC mechanism, the X-ray detector achieved high detection sensitivity. Calculated from the X-ray responsive J-E curves of the metal-semiconductor-metal-structured
Figure 3(i) shows the X-ray detection sensitivity under different X-ray tube voltages measured by a DC X-ray tube without beam filters. The sensitivity decreased quickly from
Typical temporal responses of the X-ray detectors at the electric field of
Figure 4.Temporal response of the X-ray detector. (a)–(c) Time-dependent photocurrent of the X-ray detector with pulsed X-ray source on and off at
The performance metrics of the proposed X-ray detector and literature-reported direct-conversion X-ray detectors using different photoconductors and photoelectron multiplication mechanisms are compared in Table 1. The proposed X-ray detectors formed by photoconductor targets and cold cathode field emitters can achieve high internal gain using the EBIPC mechanism. The
Comparison of Performance Metrics of Direct-Conversion X-ray Detectors Based on Different Photoconductors and Photoelectron Multiplication Mechanisms
X-ray Detector Type | Photomultiplier Mechanism | Linear Attenuation Coefficient (for 20 keV | X-ray Energy Range (keV) | Operating Electric Field ( | Dark Current ( | Photocurrent ( | Sensitivity ( | Internal Gain | Response Time (ms) | Detection Limit ( | Reference |
---|---|---|---|---|---|---|---|---|---|---|---|
EBIPC | 17.2 | 6–50 | 22.5 | 40 | 0.28 | This work | |||||
None | 17.2 | 30–60 | 0.025 | None | [ | ||||||
a-Se driven by cold cathode | Avalanche effect | 22.8 | 20–40 | 100 | Unknown | Unknown | [ | ||||
a-Se | None | 22.8 | 20–60 | 10 | 22.5 | None | [ | ||||
Perovskite ( | Photoconductive gain effect | 26.2 | 30–50 | 0.01 | 92 | [ | |||||
Perovskite ( | None | 26.2 | 20–60 | 0.005 | None | [ |
3. CONCLUSIONS
In summary, a direct-conversion vacuum cold cathode X-ray detector based on ZnO NW field emitters and
APPENDIX A: METHODS
A vacuum diode-type cold cathode X-ray detector was fabricated with the ZnO NW field emitters as the cathode and the
The morphologies of arrays of ZnO NWs were observed using a scanning electron microscope (SEM, SUPRA 60, Germany). Crystallinity of the
References
[1] Z. Z. Li, F. G. Zhou, H. H. Yao, Z. P. Ci, Z. Yang, Z. W. Jin. Halide perovskites for high-performance X-ray detector. Mater. Today, 48, 155-175(2021).
[2] G. Kakavelakis, M. Gedda, A. Panagiotopoulos, E. Kymakis, T. D. Anthopoulos, K. Petridis. Metal halide perovskites for high‐energy radiation detection. Adv. Sci., 7, 2002098(2020).
[3] M. Yaffe, J. Rowlands. X-ray detectors for digital radiography. Phys. Med. Biol., 42, 1-39(1997).
[4] S. Kasap, J. B. Frey, G. Belev, O. Tousignant, H. Mani, J. Greenspan, L. Laperriere, O. Bubon, A. Reznik, G. DeCrescenzo, K. S. Karim, J. A. Rowlands. Amorphous and polycrystalline photoconductors for direct conversion fat panel X-ray image sensors. Sensors, 11, 5112-5157(2011).
[5] Y. C. Kim, K. H. Kim, D. Son, D. Jeong, J. Seo, Y. S. Choi, I. T. Han, S. Y. Lee, N. Park. Printable organometallic perovskite enables large-area, low-dose X-ray imaging. Nature, 550, 87-91(2017).
[6] W. Pan, B. Yang, G. Niu, K. Xue, X. Du, L. Yin, M. Zhang, H. Wu, X. Miao, J. Tang. Hot-pressed CsPbBr3 quasi-monocrystalline film for sensitive direct X-ray detection. Adv. Mater., 31, 1904405(2019).
[7] B. Sinnott, E. Ron, A. B. Schneider. Exposing the thyroid to radiation: a review of its current extent, risks, and implications. Endocr. Rev., 31, 756-773(2010).
[8] R. T. Williams, W. W. Wolszczak, X. Yan, D. L. Carroll. Perovskite quantum-dot-in-host for detection of ionizing radiation. ACS Nano, 14, 5161-5169(2020).
[9] X. Y. Liu, G. Pilania, A. A. Talapatra, C. R. Stanek, B. P. Uberuaga. Band-edge engineering to eliminate radiation-induced defect states in perovskite scintillators. ACS Appl. Mater. Interfaces, 12, 46296-46305(2020).
[10] Y. Zhang, Y. Liu, Z. Xu, H. Ye, Z. Yang, J. You, M. Liu, Y. He, M. G. Kanatzidis, S. Liu. Nucleation-controlled growth of superior lead-free perovskite Cs3Bi2I9 single-crystals for high-performance X-ray detection. Nat. Commun., 11, 2304(2020).
[11] S. O. Kasap. X-ray sensitivity of photoconductors: application to stabilized a-Se. J. Phys. D, 33, 2853-2865(2000).
[12] S. O. Kasap, M. Z. Kabir, J. A. Rowlands. Recent advances in X-ray photoconductors for direct conversion X-ray image detectors. Curr. Appl. Phys., 6, 288-292(2006).
[13] Z. Su, L. E. Antonuk, M. Y. El, L. Hu, H. Du, A. Sawant, Y. Li, Y. Wang, J. Yamamoto, Q. Zhao. Systematic investigation of the signal properties of polycrystalline HgI2 detectors under mammographic, radiographic, fluoroscopic and radiotherapy irradiation conditions. Phys. Med. Biol., 50, 2907-2928(2005).
[14] Z. P. Zhang, Z. J. Zhang, W. Zheng, K. Wang, H. J. Chen, S. Z. Deng, F. Huang, J. Chen. Sensitive and fast direct conversion X-ray detectors based on single-crystalline HgI2 photoconductor and ZnO nanowire vacuum diode. Adv. Mater. Technol., 5, 1901108(2020).
[15] J. Zhao, L. Zhao, Y. Deng, X. Xiao, Z. Ni, J. Huang. Perovskite-filled membranes for flexible and large-area direct-conversion X-ray detector array. Nat. Photonics, 14, 612-617(2020).
[16] B. Yang, W. Pan, H. Wu, G. Niu, J. Yuan, K. Xue, L. Yin, X. Du, X. Miao, X. Yang, Q. Xie, J. Tang. Heteroepitaxial passivation of Cs2AgBiBr6 wafers with suppressed ionic migration for X-ray imaging. Nat. Commun., 10, 1989(2019).
[17] J. Xie, M. Chiu, E. May, Z. E. Meziani, S. Nelson, R. Wagner. MCP-PMT development at Argonne for particle identification. J. Instrum., 15, C04038(2020).
[18] B. Chen, Y. Wan, Z. Xie, J. Huang, N. Zhang, C. Shang, J. Norman, Q. Li, Y. Tong, K. M. Lau, A. C. Gossard, J. E. Bowers. Low dark current high gain InAs quantum dot avalanche photodetectors monolithically grown on Si. ACS Photon., 7, 528-533(2020).
[19] F. A. Ruffinatti, S. Lomazzi, L. Nardo, R. Santoro, A. Martemiyanov, M. Dionisi, L. Tapella, A. A. Genazzani, D. Lim, C. Distasi, M. Caccia. Assessment of a silicon-photomultiplier-based platform for the measurement of intracellular calcium dynamics with targeted aequori. ACS Sens., 5, 2388-2397(2020).
[20] D. Rui, Y. Fang, J. Chae, J. Dai, Z. Xiao, Q. Dong, Y. Yuan, A. Centrone, C. Z. Xiao, J. Huang. High-gain and low-driving-voltage photodetectors based on organolead triiodide perovskites. Adv. Mater., 27, 1912-1918(2015).
[21] Y. Honda, M. Nanba, K. Miyakawa, M. Kubota, M. Nagao, Y. Neo, H. Mimura, N. Egami. Double-gated, Spindt-type field emitter with improved electron beam extraction. IEEE Trans. Electron Dev., 63, 2182-2189(2016).
[22] T. Miyoshi, N. Igarashi, N. Matsugaki, Y. Yamada, K. Hirano, K. Hyodo, K. Tanioka, N. Egami, M. Namba, M. Kubota, T. Kawai, S. Wakatsuki. Development of an X-ray HARP–FEA detector system for high-throughput protein crystallography. J. Synchrotron Radiat., 15, 281-284(2008).
[23] Z. P. Zhang, K. Wang, K. S. Zheng, S. Z. Deng, N. S. Xu, J. Chen. Electron bombardment induced photoconductivity and high gain in a flat panel photodetector based on a ZnS photoconductor and ZnO nanowire field emitters. ACS Photon., 5, 4147-4155(2018).
[24] X. P. Bai, Z. P. Zhang, M. N. Chen, K. Wang, J. C. She, S. Z. Deng, J. Chen. Theoretical analysis and verification of electron-bombardment-induced photoconductivity in vacuum flat-panel detector. J. Lightwave Technol., 39, 2618-2624(2021).
[25] Y. F. Li, Z. P. Zhang, G. F. Zhang, L. Zhao, S. Z. Deng, N. S. Xu, J. Chen. Optimizing the field emission properties of ZnO nanowire arrays by precisely tuning the population density and application in large-area gated field emitter array. ACS Appl. Mater. Interfaces, 9, 3911-3921(2017).
[26] X. Chen, F. Ren, S. Gu, J. Ye. Review of gallium-oxide-based solar-blind ultraviolet photodetectors. Photon. Res., 7, 381-415(2019).
[27] J. Tanguay, I. A. Cunningham. Cascaded systems analysis of charge sharing in cadmium telluride photon‐counting X-ray detectors. Med. Phys., 45, 1926-1941(2018).
[28] G. Benassi, N. Zambelli, M. Villani, D. Calestani, M. Pavesi, A. Zappettini, L. Zanotti, C. Paorici. Oriented orthorhombic lead oxide film grown by vapour phase deposition for X-ray detector applications. Cryst. Res. Technol., 48, 245-250(2013).
[29] Y. B. Xu, Q. Zhou, J. Huang, W. W. Li, J. Chen, K. Wang. Highly-sensitive indirect-conversion X-ray detector with an embedded photodiode formed by a three-dimensional dual-gate thin-film transistor. J. Lightwave Technol., 38, 3775-3780(2020).
[30] Y. Gotoh, H. Tsuji, M. Nagao, T. Masuzawa, Y. Neo, H. Mimura, T. Okamoto, T. Igari, M. Akiyoshi, N. Sato, I. Takagi. Development of a field emission image sensor tolerant to gamma-ray irradiation. IEEE Trans. Electron Dev., 67, 1660-1665(2020).
[31] S. J. Young, Y. L. Chu. Characteristics of field emitters on the basis of Pd-adsorbed ZnO nanostructures by photochemical method. ACS Appl. Nano Mater., 4, 2515-2521(2021).
[32] R. H. Fowler, L. W. Nordheim. Electron emission in intense electric fields. Proc. R. Soc. London A, 119, 173-181(1928).
[33] Z. Chen, Z. Li, Y. Zhuo, W. Chen, X. Ma, Y. Pei, G. Wang. Layer-by-layer growth of ε-Ga2O3 thin film by metal-organic chemical vapor deposition. Appl. Phys. Express, 11, 101101(2018).
[35] K. A. Mengle, E. Kioupakis. Vibrational and electron-phonon coupling properties of β-Ga2O3 from first-principles calculations: impact on the mobility and breakdown field. AIP Adv., 9, 015313(2019).
[36] S. Tie, W. Zhao, D. Xin, M. Zhang, J. D. Long, Q. Chen, X. J. Zheng, J. Zhu, W. H. Zhang. Robust fabrication of hybrid lead-free perovskite pellets for stable X-ray detectors with low detection limit. Adv. Mater., 32, 2001981(2020).
[37] Z. P. Zhang, Z. M. Chen, M. N. Chen, K. Wang, H. J. Chen, S. Z. Deng, G. Hang, J. Chen. ε-Ga2O3 thin film avalanche low-energy X-ray detectors for highly sensitive detection and fast-response applications. Adv. Mater. Technol., 6, 2001094(2021).
[38] H. Liang, S. Cui, R. Su, P. Guan, Y. He, L. Yang, L. Chen, Y. Zhang, Z. Mei, X. Du. Flexible X-ray detectors based on amorphous Ga2O3 thin films. ACS Photon., 6, 351-359(2019).
[39] W. Pan, H. Wu, J. Luo, Z. Deng, C. Ge, C. Chen, X. Jiang, W. J. Yin, G. Niu, L. Zhu, L. Yin, Y. Zhou, Q. Xie, X. Ke, M. Sui, J. Tang. Cs2AgBiBr6 single-crystal X-ray detectors with a low detection limit. Nat. Photonics, 11, 726-732(2017).
[40] X. Lu, L. D. Zhou, L. Chen, X. P. Ouyang, H. Tang, B. Liu, J. Xu. X-ray detection performance of vertical Schottky photodiodes based on a bulk β-Ga2O3 substrate grown by an EFG method. ECS J. Solid State Sci. Technol., 8, Q3046-Q3049(2019).
[41] D. A. Scaduto, A. R. Lubinsky, J. A. Rowlands, H. Kenmotsu, N. Nishimoto, T. Nishino, K. Tanioka, Z. Wei. Investigation of spatial resolution and temporal performance of SAPHIRE (scintillator avalanche photoconductor with high resolution emitter readout) with integrated electrostatic focusing. Proc. SPIE, 9033, 90333S(2014).
[42] T. Masuzawa, I. Saito, T. Yamada, M. Onishi, H. Y. Yamaguchi, K. Oonuki, N. Kato, S. Ogawa, Y. Takakuwa, A. T. T. Koh, D. H. C. Chua, Y. Mori, T. Shimosawa, K. Okano. Development of an amorphous selenium-based photodetector driven by a diamond cold cathode. Sensors, 13, 13744-13778(2013).
[43] S. Abbaszadeh, C. C. Scott, O. Bubon, A. Reznik, K. S. Karim. Enhanced detection efficiency of direct conversion X-ray detector using polyimide as hole-blocking layer. Sci. Rep., 3, 3360(2013).
[44] B. Zhao, W. Zhao. Temporal performance of amorphous selenium mammography detectors. Med. Phys., 32, 128-136(2005).
[45] J. L. Peng, C. Q. Xia, Y. L. Xu, R. M. Li, L. H. Cui, J. K. Clegg, L. M. Herz, M. B. Johnston, Q. Q. Lin. Crystallization of CsPbBr3 single crystals in water for X-ray detection. Nat. Commun., 12, 1531(2021).
[46] A. Kuramata, K. Koshi, S. Watanabe, Y. Yamaoka, T. Masui, S. Yamakoshi. High-quality β-Ga2O3 single crystals grown by edge-defined film-fed growth. Jpn. J. Appl. Phys., 55, 1202A2(2016).
[47] X. Q. Cao, G. F. Zhang, Y. Y. Zhao, Y. Xu, J. C. She, S. Z. Deng, N. S. Xu, J. Chen. Fully vacuum-sealed addressable nanowire cold cathode flat-panel X-ray source. Appl. Phys. Lett., 119, 053501(2021).
[48] D. K. Chen, Y. Xu, G. F. Zhang, Z. P. Zhang, J. C. She, S. Z. Deng, N. S. Xu, J. Chen. A double-sided radiating flat-panel X-ray source using ZnO nanowire field emitters. Vacuum, 144, 266-271(2017).
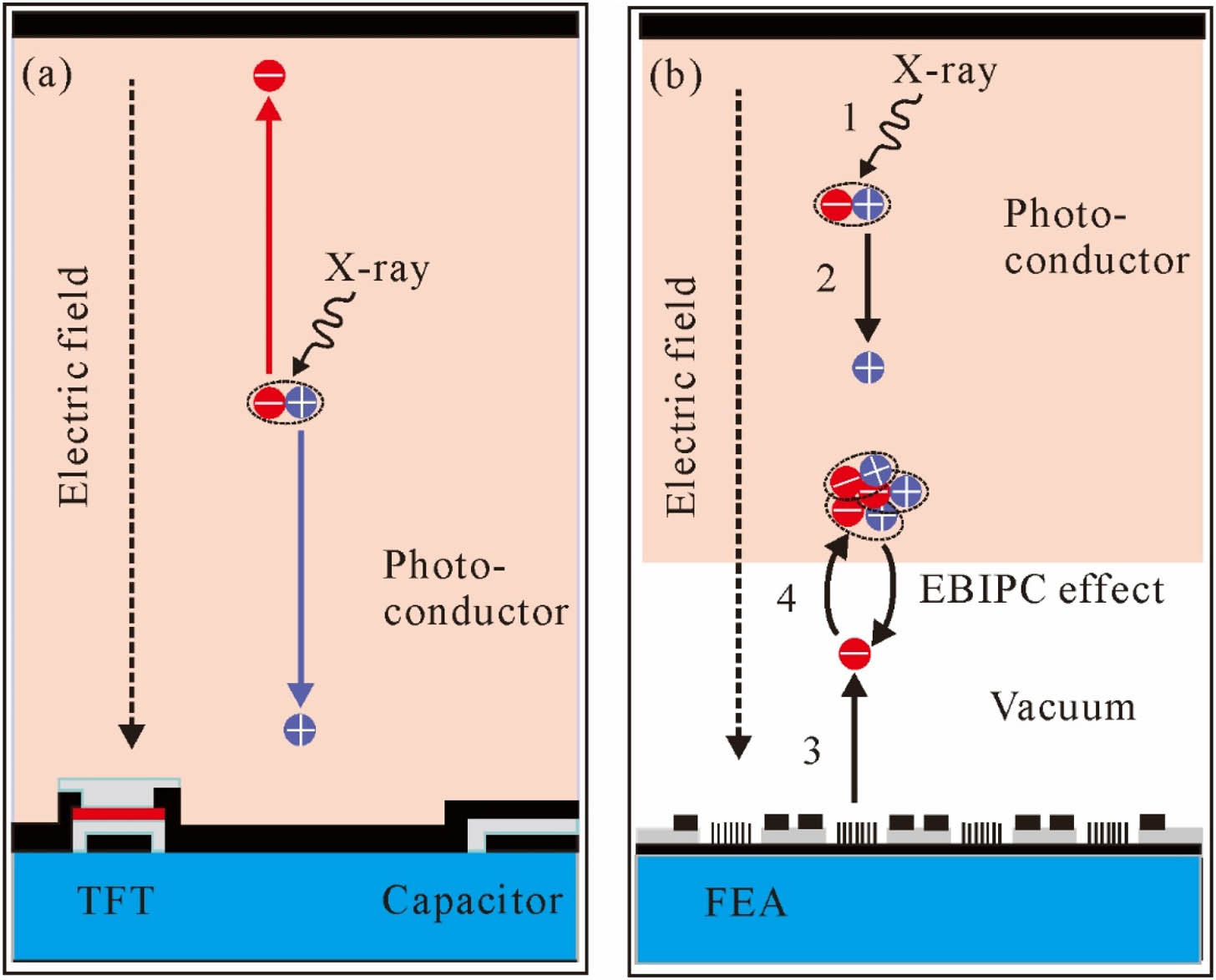
Set citation alerts for the article
Please enter your email address