Abstract
We demonstrate a passively mode-locked all-fiber laser incorporating a piece of graded-index multimode fiber as a mode-locking modulator based on a nonlinear multimodal interference technique, which generates two types of coexisting high-energy ultrashort pulses [i.e., the conventional soliton (CS) and the stretched pulse (SP)]. The CS with pulse energy as high as 0.38 nJ is obtained at the pump level of 130 mW. When the pump increases to 175 mW, the high-energy SP occurs at a suitable nonlinear phase bias and its pulse energy can reach 4 nJ at a 610 mW pump. The pulse durations of the generated CS and SP are 2.3 ps and 387 fs, respectively. The theory of nonlinear fiber optics, single-shot spectral measurement by the dispersive Fourier-transform technique, and simulation methods based on the Ginzburg–Landau equation are provided to characterize the laser physics and reveal the underlying principles of the generated CS and SP. A rogue wave, observed between the CS and SP regions, mirrors the laser physics behind the dynamics of generating a high-energy SP from a CS. The proposed all-fiber laser is versatile, cost-effective and easy to integrate, which provides a promising solution for high-energy pulse generation.1. INTRODUCTION
A temporally or spatially localized soliton can arise in numerous Kerr media resonators, stemming from the interaction between linear and nonlinear processes [1,2]. The temporal soliton formed in fiber lasers plays a vital role in telecommunications, biology, and photonics [3,4]. In soliton fiber lasers, rich passively mode-locked dynamics guide the pulse behaviors [3–6]. For example, with nonlinearity-balanced dispersion, a soliton-shaped pulse for anomalous dispersion cavity products that follows Kelly sidebands on the spectrum, is called a conventional soliton (CS) [3]. A dissipative soliton (DS) acting as a soliton-like entity will occur in a normal dispersion cavity with the existence of gain and loss [4]. However, a different pulse-shortening mechanism can coexist and compete in a resonant cavity. Because of the dispersion shift induced by a high nonlinear phase or fiber-based Lyot filter function, a dispersion-managed soliton, even a CS, can be obtained in a large normal dispersion fiber laser [5,6]. A mode-locked laser can show rich dynamic structures, which depend on the operational conditions of an optical cavity [7].
It is well known that a temporal soliton can be generated in passively mode-locked fiber lasers by combining a gain medium and a nonlinear loss element, generally a mode-locking modulator inside the resonant cavity [8–10]. To realize passively mode-locked fiber lasers, the mode-locking modulator should possess the ability of intensity discrimination, which can discriminate against the low-power signals and make high-power ones undergo minimal influence. Various technologies have been used to play the role of a mode-locking modulator, including nonlinear polarization rotation [11,12], semiconductor saturable absorber mirrors [13], carbon nanotubes [14,15], and two-dimensional (2D) materials [16–19]. Recently, the nonlinear multimodal interference (NL-MMI) technique, usually based on multimode fiber, has been proposed for ultrashort pulse generation [20–22]. Compared to traditional mode-locking modulators, an NL-MMI-based modulator possesses the potential advantages of operation for a higher peak power level and an instantaneous response time [20]. Numerous researchers have focused their efforts on NL-MMI-based fiber lasers for ultrashort and high-energy pulse generation [23–25]. Ahsan and Agrawal theoretically predicted that a fundamental graded-index soliton as short as 100 fs can form and propagate over distances beyond 1 km in multimode fiber [23]. Wang et al. proposed a 416 fs CS mode-locked fiber laser at 1591 nm with a graded-index multimode fiber (GIMF)-based mode-locking modulator, with a maximum pulse energy of 0.11 nJ [24]. Teğin and Ortaç demonstrated a DS mode-locked fiber laser at 1030 nm with a 5 ps duration and 0.13 nJ energy by combining GIMF and step-index multimode fiber [25]. To date, the pulse duration has reached the order of 100 fs; however, the pulse energy is still limited and does not go beyond lasers based on traditional modulators. Therefore, it is desirable to completely exploit the advantage of high-energy pulse generation using an NL-MMI-based mode-locking modulator.
In this paper, a passively mode-locked all-fiber laser is demonstrated based on an NL-MMI technique using GIMF, which generates a high-energy CS and stretched pulse (SP) and makes them coexist. The pulse energy of the CS reaches 0.38 nJ and that of the SP is as high as 4 nJ, which is the record of output energy using an NL-MMI technique. Meanwhile, the pulse durations of the generated CS and SP are 2.3 ps and 387 fs, respectively. The proposed all-fiber mode-locked laser is versatile and can deliver CSs and SPs, is cost-effective, and offers easy integration, which paves the way for high-energy pulse generation.
Sign up for Photonics Research TOC. Get the latest issue of Photonics Research delivered right to you!Sign up now
2. GIMF-BASED MODE-LOCKING MODULATOR
The diagrammatic sketch of a GIMF-based modulator is illustrated in Fig. 1, where the light beam is injected into a multimode fiber from a spliced single-mode fiber (SMF-28) and coupled into the other identical SMF2. Here, we only consider the radially symmetric modes () in the GIMF. When a standard single-mode light seeds a multimode fiber, a number of modes are excited and the interference between the modes occurs. In the linear limit, the transmission varies periodically along the above-mentioned structure. However, the transmission will be changed when the nonlinear effects cannot be neglected. In this case, an additional phase mismatch between the excited modes is accumulated, contributing to variations in the coupling efficiency between GIMF and SMF2, as shown in Fig. 1. Therefore, the GIMF-based modulator can discriminate against the low-power signals and slightly influence the high-power ones when the length of the GIMF is matched with a period of MMI, ( is an integer), where denotes the oscillating period because of MMI between the modes in the GIMF [20,22].

Figure 1.Schematic structure of SMF–GIMF–SMF. Blue and red lines denote light under a linear (low power) and a nonlinear (high power) case, respectively.
To verify the function of nonlinear intensity modulation, Fig. 2(a) depicts the behaviors of the transmission under our experimental conditions, which is expressed as [20] Here, and denote the total power in GIMF and the electric field amplitude of mode , respectively. To simplify the problem, we assume that the output of SMF1 only seeds the three lowest-order modes (, , and ) in the GIMF with the SMF centrally aligned with the GIMF. Figure 2(a) shows that the transmission increases to with the power increased, following with rapid oscillation before the maximum value. Figure 2(b) plots the change of relative transmission [] between the nonlinear and linear cases, which can act as a relative metric for the nonlinear modulator function. It indicates that relative transmission can be switched through fine-tuning a length of the GIMF, such as stretching the GIMF. In the tuned range of [, 300 μm], reaches its peak value with . This result is evidence that the GIMF-based structure verifies the easy integration and cost-effective mode-locking modulator in a mode-locked fiber laser.
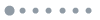
Figure 2.(a) Transmission is plotted as a function of peak power with the GIMF-length of 30 cm; (b) a relative metric for pulse power discrimination with the length of GIMF .
3. EXPERIMENTAL RESULTS AND DISCUSSION
A. Coexistence of High-Energy Mode-Locked Pulses
The GIMF-based modulator is assembled in a passively mode-locked erbium-doped fiber laser operating at a 1590 nm wavelength for high-energy pulse generation. The experimental schematic diagram of the laser is presented in Fig. 3. The gain medium is an long erbium-doped fiber (EDF). The fiber laser is pumped by two 980 nm laser diodes (LDs) with a maximum total output of 1 W, which are coupled into the cavity via a polarization-independent, tap-isolator-wavelength-division multiplexer (PI-TIWDM) and a WDM, respectively. The PI-TIWDM combines the functions of isolator, WDM, and optical coupler. The GIMF-based modulator is glued along the length direction of a polyurethane right-angled triangle with a length, width, and thickness of 31, 5, and 0.5 cm, respectively. The triangle is fixed at the optical table and the motion is controlled by a translation stage to induce a varied length by tension from 0 to 25 mm with the precision of 20 μm. The typical dispersion parameters of EDF, SMF, and GIMF are , 17, and 22 ps/(nm·km), respectively. The length of the cavity is , and the total cavity dispersion is about , which is a typical regime of SPs [6]. The 10% output extracts the laser for measurement. The left inset in Fig. 3 plots the nonlinear power-dependent transmission, expressed as , where , , and denote modulation depth, saturation intensity, and minimum transmission, respectively. The measurement gives and of and 1.8%, respectively. The right inset is the microscopy image of the GIMF-based modulator, and it clearly shows the position of splicing between GIMF and SMF.
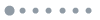
Figure 3.Experimental setup of the passively mode-locked fiber laser. Inset: nonlinear power-dependent transmission (left) and microscopy image of a GIMF-based modulator.
We note that no mode-locking operation is observed by replacing the GIMF-based modulator with an SMF of . After the modulator is inserted into the cavity, the CS is generated when the translation stage moves 14.21 mm and the pump power increases to 40 mW. When only one LD switches on and the pump increases up to 175 mW, the other distinct mode-locked pulse, the SP, can also be obtained at a suitable PC state. The typical characterizations of CSs and SPs are shown in Fig. 4, which are measured at pumps of 110 and 457 mW, respectively. As shown in Fig. 4(a), the optical spectrum of the pulse is described by the appearance of clear Kelly sidebands, which is the typical feature of CSs in an anomalous dispersion regime. Different from the CS, no sidebands are observed on the spectrum of the SP, as shown in Fig. 4(b). Note that the central wavelength of the SP (1593.3 nm) has a red-shift, compared to that of the CS (1592.8 nm). The bandwidths of the CS and SP are 6.35 and 10.54 nm, respectively. The autocorrelation trace of the CS in Fig. 4(c) indicates that the pulse duration is 2.3 ps with a fit. Figure 4(d) illustrates the autocorrelation curve of the SP and gives a pulse duration of 387 fs by fitting with a Gaussian function. The presented pulse of the CS is chirped with a time bandwidth product (TBP) and chirp parameter of 1.7 and 0.92, respectively. The TBP and chirp parameter of the SP are calculated as 0.469 and 0.24, respectively, indicating the pulse chirped slightly. The stable single-pulse operations are verified by the pulse trains with a 4 μs scan range, as shown in the left insets of Figs. 4(c) and 4(d). The corresponding radio frequency (RF) spectrum of the CS (SP) with a fundamental frequency of 5.562 MHz (5.559 MHz) is matched with the pulse separation of . The results are robust without hypermode oscillation.
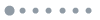
Figure 4.Characterizations of a CS and a SP. Output spectra of (a) a CS and (b) a SP plot in linear and logarithmic coordinates (insets); temporal information and RF spectra of (c) a CS and (d) a SP.
The output power and pulse energy of the laser as functions of the launched pump power are shown in Fig. 5. When the pump power locates in the range of 25–130 mW, the CS can stably exist in the cavity. The pulse energy of the CS increases near linearly with the pump power in region A, and the maximum pulse energy of the CS is 0.38 nJ at a pump of 130 mW. To further increase the pump power, the operation of the CS transforms into the multipulse state (region B). When the PC state is varied, the polarization-dependent loss causes pulse energy fluctuations [7,26,27], and the SP pulse rises at a pump above 175 mW. Considering that the GIMF-based modulator plays a role in spectral filtering, the pulse chirp varies with operational conditions [28–30]. With the pump in the range of 175–610 mW, the SP pulse can stably operate in the optical cavity at a suitable PC state. We note that the slope efficiency varies when the pump is above 500 mW, which results from the second LD switched on and the accuracy difference between the two LDs. In region C, a maximum average output power of 22 mW is achieved at a 610 mW pump, and the corresponding pulse energy is as high as 4 nJ, which is higher than most of the 1D and 2D material mode-locked fiber lasers. Then, with the pump power increasing, the SP becomes multipulse.
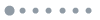
Figure 5.Output power (blue) and pulse energy (red) as functions of launched pump power. A, B, C, and D denote the regions of the laser operations for generating a CS, the multipulse state of the CS, a SP, and the multipulse state of the SP, respectively. The slope efficiencies of the laser in regions A and C are and , respectively.
As we all know, the CS energy is limited by the soliton area theorem, and is inversely proportional to the nonlinearity of the optical resonator. Thus, the soliton energy is limited to in standard fiber [4]. However, in our experiment, the CS energy can reach 0.38 nJ, which is about fourfold higher than the theoretical limit. First, the large core diameter and smaller nonlinear refractive index () of GIMF can decrease the nonlinear coefficient of the optical resonator. Second, the GIMF-based structure has the advantage of operating at a much higher power strength [20], and it performs the sufficient role of modulating laser along a -cm-length GIMF, which can be seen in Fig. 2. In our experiment, the mode-locking modulator has a high damage threshold of . Therefore, the high-energy pulses (i.e., the CS and SP), can be obtained in our fiber laser.
B. Mechanism of Mode-Locking Transition
Because the CS is chirped with , the total cavity dispersion under CS operation can be obtained by [31] Here, , , , and denote the frequency separation from the central frequency to the m-order sideband, the average small-signal gain coefficient over the cavity length, the total length of the cavity, and the curvature of the gain spectrum at the lasing wavelength, respectively. Under CS operation, the total cavity dispersion is calculated as , which is much large than the value of . This phenomenon can be expressed by the modal group delay, which is a key property governing the modal dispersion of a pulse in GIMF. For the -law GIMF, (calculated as 2.1007) is chosen to minimize the multimode dispersion, and the group delay dispersion between modes is small for angularly symmetric modes () [32]. Therefore, the group delay induced by the principal modes (PMs) excited in GIMF is the key factor that results in this phenomenon, and the PMs show the polarization sensitivity and linear dependence between group delay and short GIMF length [33]. The bandwidth BW of the filter induced by PC and a given continuous bend in GIMF is expressed as [34], where denotes the phase delay between PMs in GIMF. As discussed above, the dispersion for the CS () is larger than for the SP (), which means that the dispersion-managed soliton possesses a relatively small phase delay . In other words, the optical bandwidth of the SP is larger than the CS, which agrees well with our experiments.
Therefore, the mode-locking operations’ transition from the CS to the SP regime is attributed to the interaction of the dispersion and complicated nonlinear effects in GIMF. When the propagation constant mismatch between modes is larger than the average inverse nonlinear length under low pump power, the CS is generated in the anomalous dispersion region () with a suitable PC state. When the pulse peak power increases to a certain value, the accumulated temporal walk-off leads to a decrease in the modulation depth and bandwidth of the filter, and the excess nonlinearity results in collapse of the CS operation. With the pump increased further, the operation steps into the near-zero dispersion regime (), as long as the modal group delay resulting from PMs is suppressed by the PC in the laser cavity. In this case, the parametric processes provide a large gain and the intermodal four-wave mixing is suppressed, governed by a single beat length, where the stability of the SP is improved, as shown by the RF spectra in Figs. 4(c) and 4(d).
To gain insight into the two types of dynamic evolutions, we observe their real-time spectra by the dispersive Fourier-transform (DFT) technique. The detailed measure method can be seen from our previous work [10]. The length of dispersion-compensating fiber (DCF) used in the experiment is , which supports a total group delay dispersion of . Figure 6 plots several discontinuous spectra from real-time spectral measurements of CS and SP. We can see a phase of spectral broadening before mode-locking, such as Fig. 6(b). From Fig. 6(a), we find that a distinct sideband centers at , corresponding to that of Fig. 4(a). Because of the loss of DCF and the narrow bandwidth of the sidebands, the sidebands measured by DFT decrease greatly in amplitude. The displayed data sets show us some main information. First, there are no signs of overshoot events in the intracavity energy during mode-locking transition, which is quite different from Herink’s observations [35]. This phenomenon indicates that the NL-MMI resulting from an SMF–GIMF–SMF structure arrests the temporal walk-off between pulses by altering the transient energy in the GIMF-based modulator, which confirms the explanation above. Second, we note that the spent time in the transient process of a CS pulse is greater than that of a SP (about 6000 roundtrips; i.e., ), which indicates that the rate of selecting modes and suppression of perturbation are different between a CS and a SP. Furthermore, we find that the spectra broaden rapidly among the transient process of a SP, being distinct from that of a CS in Fig. 6(a), which goes through a phase of a metastable state with , and then the spectral bandwidth broadens and the energy rises to reach stability. In the inset of Fig. 6(a), spectral breathing can be seen among the metastable state, which indicates the soliton power instability in this regime.
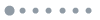
Figure 6.Real-time observations of spectral dynamics of (a) a CS and (b) a SP. Inset: continuous real-time spectra measured by the DFT.
A chaos regime exists between the CS and SP regimes in our experiment, as shown in Fig. 7, which plays a vital role in the generation of high-energy pulses. A rogue wave is a pulse of huge peak power, which can chaotically appear in the laser cavity [36]. It is the main mechanism for generating a rogue wave that transfers the background energy into a single strong wave, and a rogue wave is one of the ultimate methods to govern the generation of a high-energy pulse in modern optics, especially in the generation of high-energy SP from lower-energy CS in our experiment [37]. The observation of a rogue wave in our experiments mirrors laser physics behind the formation of a high-energy SP, which provides a new insight into the generation of a high-energy pulse. Figure 7 shows the measurements of a rogue wave by the DFT technique at the transition regime, which considers 100,000 roundtrips of pulse dynamics for the statistical distribution. Figure 7 indicates some features. First, the biggest rogue pulses (intensity of 2.5) exceed the significant wave height (SWH, 0.99) by a factor of ; second, the rogue wave appears and disappears spontaneously, as shown in the inset of Fig. 7; and third, the statistical distribution is L-shaped with long tails [36,37].
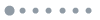
Figure 7.Statistical information and the dynamics process of a rogue wave.
To gain a broader understanding of our experimental phenomenon and modify the computational demands, we numerically simulate the evolution of passively mode-locked pulses in fiber lasers using the Ginzburg–Landau equation [6,38]. Considering the spectral filtering of GIMF [25,28], we add a spectral filter in the simulation. In the simulation, we select a -type transfer function to model the mode-locked modulator, matching with the measured results. Considering the coupling efficiency, we add the linear loss to the model. Our numerical results show that two types of pulses can coexist and compete in a mode-locked fiber laser. With a pump strength of , the SP with a narrow pulse width is obtained, as shown in Fig. 8(b). When the cavity loss and pump level are varied, the function of spectral filtering weakens and the soliton formation reshapes the pulse into a chirped pulse, which is plotted in Fig. 8(a). Figures 8(c) and 8(d) plot the intensity evolutions of the CS and SP along the roundtrip, respectively. The rates of spectral broadening are different between the CS and SP. Because of the relatively low amplitude of the sidebands, they are invisible from the CS evolution process in the linear coordinate. Figure 8(c) reveals that the oscillation of spectral bandwidth exists before the stable state, corresponding to the metastable state in the inset of Fig. 6(a).
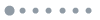
Figure 8.Numerical simulation results. Temporal and spectral profiles of (a) a CS and (b) a SP; the spectral evolution of (c) CS and (d) SP pulses.
4. CONCLUSION
To summarize, a piece of GIMF is inserted in an all-fiber laser for passively mode-locking based on an NL-MMI technique, which generates a coexisting high-energy CS and SP. The pulse energy of the CS reaches 0.38 nJ at 130 mW pump power, and that of the SP is as high as 4 nJ at a 610 mW pump. The pulse durations of the generated CS and SP are 2.3 ps and 387 fs, respectively, and the corresponding spectral bandwidths are 6.35 and 10.54 nm, respectively. Because of the functions of spectral filtering in the GIMF-based modulator, the bandwidth of the filter varies with the pump level and loss in the cavity, helping reshape the pulse into the other type and changing the chirp of the pulse. The experimental and simulation results reveal that two types of dynamic evolutions coexist in the laser cavity. The presented mode-locked all-fiber laser shows a high damage threshold , resulting from the advantage of GIMF. Compared to a recent report [39], the rogue wave observed in our experiments mirrors the laser physics behind the formation of high-energy SP, which provides a new insight into the generation of a high-energy pulse. This cost-effective, all-fiber mode-locked laser offers easy integration and is versatile enough to deliver a CS and a SP, which would play a vital role in high-energy pulse generation.
References
[1] W. H. Renninger, F. W. Wise. Optical solitons in graded-index multimode fibers. Nat. Commun., 4, 1719(2013).
[2] P. M. W. French. The generation of ultrashort laser pulses. Rep. Prog. Phys., 58, 169-262(1995).
[3] H. Zhang, Q. Bao, D. Tang, L. Zhao. Large energy soliton erbium-doped fiber laser with a graphene-polymer composite mode locker. Appl. Phys. Lett., 95, 141103(2009).
[4] F. W. Wise, A. Chong, W. H. Renninger. High-energy femtosecond fiber lasers based on pulse propagation at normal dispersion. Laser Photon. Rev., 2, 58-73(2008).
[5] L. Yun. Generation of vector dissipative and conventional solitons in large normal dispersion regime. Opt. Express, 25, 18751-18759(2017).
[6] X. Wu, D. Y. Tang, L. M. Zhao, H. Zhang. Effective cavity dispersion shift induced by nonlinearity in a fiber laser. Phys. Rev. A, 80, 013804(2009).
[7] S. Wang, A. Docherty, B. S. Marks, C. R. Menyuk. Boundary tracking algorithms for determining the stability of mode-locked pulses. J. Opt. Soc. Am. B, 31, 2914-2930(2014).
[8] D. Mao, X. Cui, X. Gan, M. Li, W. Zhang, H. Lu, J. Zhao. Passively Q-switched and mode-locked fiber laser based on a ReS2 saturable absorber. IEEE J. Sel. Top. Quantum Electron., 24, 1100406(2018).
[9] L. F. Mollenauer, R. H. Stolen, J. P. Gordon. Experimental observation of picosecond pulse narrowing and solitons in optical fibers. Phys. Rev. Lett., 45, 1095-1098(1980).
[10] G. Wang, G. Chen, W. Li, C. Zeng, H. Yang. Decaying evolution dynamics of double-pulse mode-locking. Photon. Res., 6, 825-829(2018).
[11] D. Y. Tang, L. M. Zhao, X. Wu, H. Zhang. Soliton modulation instability in fiber lasers. Phys. Rev. A, 80, 023806(2009).
[12] S. Hu, J. Yao, M. Liu, A. P. Luo, Z. C. Luo, W. C. Xu. Gain-guided soliton fiber laser with high-quality rectangle spectrum for ultrafast time-stretch microscopy. Opt. Express, 24, 10786-10796(2016).
[13] L. M. Zhao, D. Y. Tang, X. Wu, H. Zhang, H. Y. Tam. Coexistence of polarization-locked and polarization-rotating vector solitons in a fiber laser with SESAM. Opt. Lett., 34, 3059-3061(2009).
[14] M. Liu, A. P. Luo, Z. C. Luo, W. C. Xu. Dynamics trapping of a polarization rotation vector soliton in a fiber laser. Opt. Lett., 42, 330-333(2017).
[15] M. Chernysheva, A. Rozhin, Y. Fedotov, C. Mou, R. Arif, S. M. Kobtsev, E. M. Dianov, S. K. Turitsyn. Carbon nanotubes for ultrafast fiber lasers. Nanophotonics, 6, 1-30(2017).
[16] L. Yun. Black phosphorus saturable absorber for dual-wavelength polarization-locked vector soliton generation. Opt. Express, 25, 32380-32385(2017).
[17] J. Liu, Y. Chen, Y. Li, H. Zhang, S. Zheng, S. Xu. Switchable dual-wavelength Q-switched fiber laser using multilayer black phosphorus as a saturable absorber. Photon. Res., 6, 198-203(2018).
[18] D. Mao, B. Du, D. Yang, S. Zhang, Y. Wang, W. Zhang, X. She, H. Cheng, H. Zeng, J. Zhao. Nonlinear saturable absorption of liquid-exfoliated molybdenum/tungsten ditelluride nanosheets. Small, 12, 1489-1497(2016).
[19] Y. Ge, Z. Zhu, Y. Xu, Y. Chen, S. Chen, Z. Liang, Y. Song, Y. Zou, H. Zeng, S. Xu, H. Zhang, D. Fan. Broadband nonlinear photoresponse of 2D TiS2 for ultrashort pulse generation and all-optical thresholding devices. Adv. Opt. Mater., 6, 1701166(2018).
[20] E. Nazemosadat, A. Mafi. Nonlinear multimodal inference and saturable absorber using a short graded-index multimode optical fiber. J. Opt. Soc. Am. B, 30, 1357-1367(2013).
[21] H. Li, Z. Wang, C. Li, J. Zhang, S. Xu. Mode-locked Tm fiber laser using SMF-SIMF-GIMF-SMF fiber structure as a saturable absorber. Opt. Express, 25, 26546-26553(2017).
[22] F. Poletti, P. Horak. Description of ultrashort pulse propagation in multimode optical fibers. J. Opt. Soc. Am. B, 25, 1645-1654(2008).
[23] A. S. Ahsan, G. P. Agrawal. Graded-index solitons in multimode fibers. Opt. Lett., 43, 3345-3348(2018).
[24] Z. Wang, D. N. Wang, F. Wang, L. Li, C. Zhao, B. Xu, S. Jin, S. Cao, Z. Fang. Stretched graded-index multimode optical fiber as a saturable absorber for erbium-doped fiber laser mode locking. Opt. Lett., 43, 2078-2081(2018).
[25] U. Teğin, B. Ortaç. All-fiber all-normal dispersion femtosecond laser with a nonlinear multimodal interference-based saturable absorber. Opt. Lett., 43, 1611-1614(2018).
[26] Y. Shen, J. Zweck, S. Wang, C. R. Menyuk. Spectra of short pulse solutions of the cubic-quintic complex Ginzburg Landau equation near zero dispersion. Stud. Appl. Math., 137, 238-255(2016).
[27] C. Bao, W. Chang, C. Yang, N. Akhmediev, S. T. Cundiff. Observation of coexisting dissipative solitons in a mode-locked fiber lasers. Phys. Rev. Lett., 115, 253903(2015).
[28] A. Mafi, P. Hofmann, C. J. Salvin, A. Schülzgen. Low-loss coupling between two single-mode optical fibers with different mode-field diameters using a graded-index multimode optical fiber. Opt. Lett., 36, 3596-3598(2011).
[29] B. Oktem, C. Ülgüdür, F. Ö. IIday. Soliton-similariton fiber laser. Nat. Photonics, 4, 307-311(2010).
[30] A. Chong, W. H. Renninger, F. W. Wise. Properties of normal-dispersion femtosecond fiber laser. J. Opt. Soc. Am. B, 25, 140-148(2008).
[31] L. W. Liou, G. P. Agrawal. Effect of frequency chirp on soliton spectral sidebands in fiber lasers. Opt. Lett., 20, 1286-1288(1995).
[32] A. Mafi. Pulse propagation in a short nonlinear graded-index multimode optical fiber. J. Lightwave Technol., 30, 2803-2811(2012).
[33] M. B. Shemirani, W. Mao, R. A. Panicker, J. M. Kahn. Principal modes in graded-index multimode fiber in presence of spatial- and polarization-mode coupling. J. Lightwave Technol., 27, 1248-1261(2009).
[34] K. Özgören, F. Ö. IIday. All-fiber all-normal dispersion laser with a fiber-based Lyot filter. Opt. Lett., 35, 1296-1298(2010).
[35] G. Herink, B. Jalali, C. Ropers, D. R. Solli. Resolving the build-up of femtosecond mode-locking with single-shot spectroscopy at 90 MHz frame rate. Nat. Photonics, 10, 321-326(2016).
[36] M. Onorato, S. Residori, U. Bortolozzo, A. Montina, F. T. Arecchi. Rogue waves and their generating mechanisms in different physical contexts. Phys. Rep., 528, 47-89(2013).
[37] D. R. Solli, C. Ropers, P. Koonath, B. Jalali. Optical rogue waves. Nature, 450, 1054-1057(2007).
[38] D. D. Han. Experimental and theoretical investigations of a tunable dissipative soliton fiber laser. Appl. Opt., 53, 7629-7633(2014).
[39] F. Zhao, Y. Wang, H. Wang, Z. Yan, X. Hu, W. Zhang, T. Zhang, K. Zhou. Ultrafast soliton and stretched-pulse switchable mode-locked fiber laser with hybrid structure of multimode fiber-based saturable absorber. Sci. Rep., 8, 16369(2018).