
- Chinese Optics Letters
- Vol. 20, Issue 4, 042201 (2022)
Abstract
1. Introduction
Localized surface plasmons[
With the above appealing physical properties, graphene plasmons are promising to construct future compact sensing devices[
To overcome the limitations mentioned above, in this work, a three-level plasmonic system made of four rotationally aligned graphene strips is proposed for high-resolution and multi-line[
Sign up for Chinese Optics Letters TOC. Get the latest issue of Chinese Optics Letters delivered right to you!Sign up now
2. Structure and Design
The aim of our design is to produce a polarization-insensitive multi-line spectrum with extensive localized field area. Figure 1(a) describes the graphene metasurface fabricated on a quartz substrate, and the unit cell is composed of four rotationally arranged graphene strips, where the periods of the unit cell along the
Figure 1.(a) Schematic view of the proposed graphene metasurface, where the width of graphene strip w = 300 nm, length a = 650 nm, period L = 900 nm, and the polarization is along the x axis. (b) Dispersion map of absorption with different spacings d offset by unity for clarity. (c) Simulated absorption spectra as a function of separation d between graphene strips and frequency.
To explore the plasmon hybridization effect, finite difference time domain (FDTD)[
Figure 2.Localized field distribution 2D plot at 38 THz with different spacings: (a) d = 150 nm, (b) d = 100 nm, and (c) d = 50 nm in the hybridized mode. (d) Indication of the electric dipole (ED) moment and the orthogonal magnetic dipole (MD) moment involved with the super-radiant state and the quasi BIC, respectively.
3. Results and Discussions
To quantitatively describe the hybridization process of the metasurface, which consists of the artificial states of the super-radiant state [
The amplitudes of both states exhibit EIT performance. In the analogue of the metastable level of an atom, as described in Fig. 3(b), the dipole-allowed transition process between the super-radiant mode and light is from
Figure 3.(a) Q-factor and band structure of BIC mode in the graphene metasurface. (b) Level scheme for the resonances in a schematic three-level system. The super-radiant mode is indicated by blue and the BIC-based mode by green.
Figure 4.Absorption spectra and localized field distribution at resonances around 38 THz. (a) Symmetry-protected BIC composed of vertically aligned graphene strips. (b) Quasi BIC via breaking symmetry. (c) Super-radiant (bright) mode composed of horizontal graphene strips. (d) Three-level system for multi-line spectrum via hybridization.
Starting from the symmetric configuration with
To further understand the system robustness to the relaxation time induced by the concentration of defective impurities, simulations for the optical spectra are performed based on the FDTD algorithm. As illustrated in Fig. 5(a), when the Fermi energy level is fixed at 0.6 eV, as the relaxation time (
Figure 5.(a) Absorption spectra versus different relaxation times. (b), (c) Dispersion maps with different lengths and widths of graphene strips. The separation between graphene strips is 45 nm.
Apart from relaxation time and structural feature, the effect of incident polarization is also investigated. Changing the polarization angle from 0° to 45°, the spectra as plotted in Fig. 6 show that the designed structure is immune to the incident polarization, which is important in practical wide-angle settings.
Figure 6.Simulated absorption spectra as a function of polarization angle ϕ and frequency with 45 nm separation between graphene strips.
4. Conclusion
In conclusion, by exploiting the mechanism of plasmon hybridization induced by quasi BIC, a high-Q polarization-insensitive graphene metasurface oriented for sensing in two dimensions is designed. The effective area of the localized electromagnetic field is significantly enlarged via hybridizing different resonance modes, resulting in multi-line spectra in a three-level system, which is immune to the polarization angle of incident light. In addition, the robustness to relaxation time and the effect of structural features on the spectra are also calculated. The proposed graphene-based structure holds great potential for developing compact nanoscale devices with two-dimensional high sensing capacity.
References
[1] J. You, X. Li, F. Xie, W. E. I. Sha, J. H. W. Kwong, G. Li, W. C. H. Choy, Y. Yang. Surface plasmon and scattering-enhanced low-bandgap polymer solar cell by a metal grating back electrode. Adv. Energy Mater., 2, 1203(2012).
[2] S. Sun, H. Chen, W. Zheng, G. Guo. Dispersion relation, propagation length and mode conversion of surface plasmon polaritons in silver double-nanowire systems. Opt. Express, 21, 14591(2013).
[3] Y. Zhan, Y. Li, Z. Wu, S. Hu, Z. Li, X. Liu, J. Yu, Y. Huang, G. Jing, H. Lu, H. Guan, W. Qiu, J. Dong, W. Zhu, J. Tang, Y. Luo, J. Zhang, Z. Chen. Surface plasmon resonance-based microfiber sensor with enhanced sensitivity by gold nanowires. Opt. Mater. Express, 8, 3927(2018).
[4] Y. Zheng, H. Liu, J. Li, J. Xiang, M. Panmai, Q. Dai, Y. Xu, S. Tie, S. Lan. Controllable formation of luminescent carbon quantum dots mediated by the Fano resonances formed in oligomers of gold nanoparticles. Adv. Mater., 31, 1901371(2019).
[5] M. Olszyna, A. Debrassi, C. Üzüm, L. Dähne. Label-free bioanalysis based on low-Q whispering gallery modes: rapid preparation of microsensors by means of layer-by-layer technology. Adv. Funct. Mater., 29, 1805998(2019).
[6] Y. Kuai, J. Chen, X. Tang, Y. Xiang, F. Lu, C. Kuang, L. Xu, W. Shen, J. Cheng, H. Gui, G. Zou, P. Wang, H. Ming, J. Liu, X. Liu, J. R. Lakowicz, D. Zhang. Label-free surface-sensitive photonic microscopy with high spatial resolution using azimuthal rotation illumination. Sci. Adv., 5, v5335(2019).
[7] F. Wang, B. Chen, B. Yan, Y. Yin, L. Hu, Y. Liang, M. Song, G. Jiang. Scattered light imaging enables real-time monitoring of label-free nanoparticles and fluorescent biomolecules in live cells. J. Am. Chem. Soc., 141, 14043(2019).
[8] N. Liu, M. Mesch, T. Weiss, M. Hentschel, H. Giessen. Infrared perfect absorber and its application as plasmonic sensor. Nano Lett., 10, 2342(2010).
[9] N. Liu, T. Weiss, M. Mesch, L. Langguth, U. Eigenthaler, M. Hirscher, C. Sönnichsen, H. Giessen. Planar metamaterial analogue of electromagnetically induced transparency for plasmonic sensing. Nano Lett., 10, 1103(2010).
[10] H. Tayoub, A. Hocini, A. Harhouz. High-sensitive mid-infrared photonic crystal sensor using slotted-waveguide coupled-cavity. Prog. Electromagn. Res. M, 105, 45(2021).
[11] Y. Qin, Y. Fang, L. Wang, S. Tang, S. Sun, Z. Liu, Y. Mei. Surface wave resonance and chirality in a tubular cavity with metasurface design. Opt. Commun., 417, 42(2018).
[12] F. Guan, S. Sun, S. Ma, Z. Fang, B. Zhu, X. Li, Q. He, S. Xiao, L. Zhou. Transmission/reflection behaviors of surface plasmons at an interface between two plasmonic systems. J. Condens. Matter Phys., 30, 114002(2018).
[13] J. Li, Y. Xu, Q. Dai, S. Lan, S. Tie. Manipulating light-matter interaction in a gold nanorod assembly by plasmonic coupling. Laser Photonics Rev., 10, 826(2016).
[14] W. Sun, Q. He, S. Sun, L. Zhou. High-efficiency surface plasmon meta-couplers: concept and microwave-regime realizations. Light Sci. Appl., 5, e16003(2016).
[15] S. Sun, Q. He, J. Hao, S. Xiao, L. Zhou. Electromagnetic metasurfaces: physics and applications. Adv. Opt. Photonics, 11, 380(2019).
[16] J. W. You, N. C. Panoiu. Plasmon-induced nonlinearity enhancement and homogenization of graphene metasurfaces. Opt. Lett., 44, 3030(2019).
[17] X. Yan, M. Yang, Z. Zhang, L. Liang, D. Wei, M. Wang, M. Zhang, T. Wang, L. Liu, J. Xie, J. Yao. The terahertz electromagnetically induced transparency-like metamaterials for sensitive biosensors in the detection of cancer cells. Biosens. Bioelectron., 126, 485(2019).
[18] C. Wang, X. Jiang, G. Zhao, M. Zhang, C. W. Hsu, B. Peng, A. D. Stone, L. Jiang, L. Yang. Electromagnetically induced transparency at a chiral exceptional point. Nat. Phys., 16, 334(2020).
[19] J. Zhong, X. Xu, Y. Lin. Tunable terahertz metamaterial with electromagnetically induced transparency characteristic for sensing application. Nanomaterials, 11, 2175(2021).
[20] N. P. Montoni, S. C. Quillin, C. Cherqui, D. J. Masiello. Tunable spectral ordering of magnetic plasmon resonances in noble metal nanoclusters. ACS Photonics, 5, 3272(2018).
[21] A. V. Kabashin, P. Evans, S. Pastkovsky, W. Hendren, G. A. Wurtz, R. Atkinson, R. Pollard, V. A. Podolskiy, A. V. Zayats. Plasmonic nanorod metamaterials for biosensing. Nat. Mater., 8, 867(2009).
[22] C. Gong, M. S. Leite. Noble metal alloys for plasmonics. ACS Photonics, 3, 507(2016).
[23] D. Z. Manrique, J. W. You, H. Deng, F. Ye, N. C. Panoiu. Quantum plasmon engineering with interacting graphene nanoflakes. J. Phys. Chem. C, 121, 27597(2017).
[24] Q. Ren, J. W. You, N. Panoiu. Comparison between the linear and nonlinear homogenization of graphene and silicon metasurfaces. IEEE Access, 8, 175753(2020).
[25] Q. Ren, J. W. You, N. C. Panoiu. Large enhancement of the effective second-order nonlinearity in graphene metasurfaces. Phys. Rev. B, 99, 205404(2019).
[26] Z. Lan, J. W. You, Q. Ren, W. E. I. Sha, N. C. Panoiu. Second-harmonic generation via double topological valley-Hall kink modes in all-dielectric photonic crystals. Phys. Rev. A, 103, L041502(2021).
[27] X. Xiong, Y. Chen, H. Wang, S. Hu, Y. Luo, J. Dong, W. Zhu, W. Qiu, H. Guan, H. Lu, J. Yu, J. Zhang, Z. Chen. Plasmonic interface modified with graphene oxide sheets overlayer for sensitivity enhancement. ACS Appl. Mater. Interfaces, 10, 34916(2018).
[28] R. Wang, X. Ren, Z. Yan, L. Jiang, W. E. I. Sha, G. Shan. Graphene based functional devices: a short review. Front. Phys., 14, 13603(2019).
[29] Y. Yin, J. Pang, J. Wang, X. Lu, Q. Hao, E. Saei Ghareh Naz, X. Zhou, L. Ma, O. G. Schmidt. Graphene-activated optoplasmonic nanomembrane cavities for photodegradation detection. ACS Appl. Mater. Interfaces, 11, 15891(2019).
[30] Y. Qin, X. Xiong, W. Sha, L. J. Jiang. Electrically tunable polarizer based on graphene-loaded plasmonic cross antenna. J. Phys. Condens. Matter, 30, 144007(2018).
[31] Y. P. Chen, W. E. I. Sha, L. Jiang, J. Hu. Graphene plasmonics for tuning photon decay rate near metallic split-ring resonator in a multilayered substrate. Opt. Express, 23, 2798(2015).
[32] X. Ren, W. E. I. Sha, W. C. H. Choy. Tuning optical responses of metallic dipole nanoantenna using graphene. Opt. Express, 21, 31824(2013).
[33] Q. Ren, F. Feng, X. Yao, Q. Xu, M. Xin, Z. Lan, J. You, X. Xiao, W. E. I. Sha. Multiplexing-oriented plasmon-MoS2 hybrid metasurfaces driven by nonlinear quasi bound states in the continuum. Opt. Express, 29, 5384(2021).
[34] Z. Zhang, F. Qin, Y. Xu, S. Fu, Y. Wang, Y. Qin. Negative refraction mediated by bound states in the continuum. Photonics Res., 9, 1592(2021).
[35] J. Xiang, Y. Xu, J. Chen, S. Lan. Tailoring the spatial localization of bound state in the continuum in plasmonic-dielectric hybrid system. Nanophotonics, 9, 133(2020).
[36] Q. Ren, J. W. You, N. C. Panoiu. Giant enhancement of the effective Raman susceptibility in metasurfaces made of silicon photonic crystal nanocavities. Opt. Express, 26, 30383(2018).
[37] X. Wang, J. Ma, Q. Ren, M. Wang, Z. Yang, J. Xin. Effects of Fe3+-doping and nano-TiO2/WO3 decoration on the ultraviolet absorption and gas-sensing properties of ZnSnO3 solid particles. Sens. Actuators B, 344, 130223(2021).
[38] Z. Liu, Y. Xu, Y. Lin, J. Xiang, T. Feng, Q. Cao, J. Li, S. Lan, J. Liu. High-Q quasibound states in the continuum for nonlinear metasurfaces. Phys. Rev. Lett., 123, 253901(2019).
[39] H. Hao, S. Zheng, Y. Tang, X. Ran. Design of electromagnetic wave multi-type focusing based on 1-bit metasurface. Prog. Electromagn. Res. M, 105, 79(2021).
[40] Q. Fu, Q. Feng, H. Chen. Design and optimization of CPW-fed broadband circularly polarized antenna for multiple communication systems. Prog. Electromagn. Res. M, 99, 65(2021).
[41] X. Lai, Q. Ren, F. Vogelbacher, W. E. I. Sha, X. Hou, X. Yao, Y. Song, M. Li. Bioinspired quasi-3D multiplexed anti-counterfeit imaging via self-assembled and nanoimprinted photonic architectures. Adv. Mater., 34, 2107243(2021).
[42] R. Hao, E. Cassan, Y. Xu, M. Qiu, X. Wei, E. Li. Reconfigurable parallel plasmonic transmission lines with nanometer light localization and long propagation distance. IEEE J. Sel. Top. Quantum Electron., 19, 4601809(2013).
[43] G. Calvaruso, A. Zaeim. On the symmetries of the Lorentzian oscillator group. Collect. Math., 68, 51(2017).
[44] J. Applequist, K. R. Sundberg, M. L. Olson, L. C. Weiss. A normal mode treatment of optical properties of a classical coupled dipole oscillator system with Lorentzian band shapes. J. Chem. Phys., 70, 1240(1979).
[45] E. Ott, T. M. Antonsen. Low dimensional behavior of large systems of globally coupled oscillators. Chaos, 18, 37113(2008).
[46] F. Chen, K. Li. One-step absolutely stable FDTD methods for electromagnetic simulation. Prog. Electromagn. Res., 100, 45(2021).
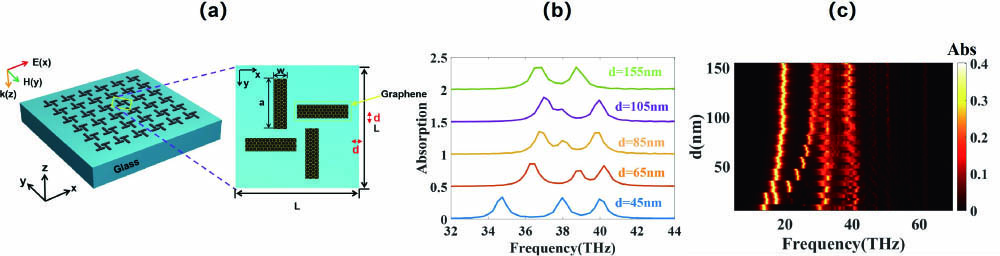
Set citation alerts for the article
Please enter your email address