Andrey S. Sokolov, Haider Abbas, Yawar Abbas, Changhwan Choi. Towards engineering in memristors for emerging memory and neuromorphic computing: A review[J]. Journal of Semiconductors, 2021, 42(1): 013101

Search by keywords or author
- Journal of Semiconductors
- Vol. 42, Issue 1, 013101 (2021)
![(Color online) Recent materials used for memristive storage and applied in artificial synapses. (a) The sketch of filamentary-based resistive switching in an inorganic metal oxide (HfOx) memristor. Reprinted from Ref. [41]. (b) Schematic of inorganic metal oxide-based hetero-structured device, as Al/Al2O3/NbxOy/Au device. Reprinted from Ref. [52]. (c) Chemical diagram and schematic of polymer-based PVK-C60 memristive device. Reprinted from Ref. [58]. (d) Naturally extracted nanocellulose-based memristive device. Reprinted from Ref. [65]. (e) 2-dimensional (2D) shape of graphene. Reprinted from Ref. [71]. (f) 2D material MoS2, sandwiched between graphene electrode-based memristive device. Reprinted from Ref. [61]. (g) 3-terminal memristive device based on WSe2 2D material. Reprinted from Ref. [64]. (h) Schematic of PVPy-doped Au@Ag bimetal nanoparticle memristor storage. Reprinted from Ref [72]. (i) Sketch of an Ag/MAPbI3/FTO organic-inorganic hybrid perovskite artificial synapse device. Reprinted from Ref. [32], (j) Graphene oxide (GO) quantum dots (QDs) -based memristive storage device. Reprinted from Ref. [73], (k) InP/ZnS core-shell QDs-based memristive device, applied as an artificial synapse. Reprinted from Ref. [74].](/richHtml/jos/2021/42/1/013101/img_1.jpg)
Fig. 1. (Color online) Recent materials used for memristive storage and applied in artificial synapses. (a) The sketch of filamentary-based resistive switching in an inorganic metal oxide (HfOx ) memristor. Reprinted from Ref. [41 ]. (b) Schematic of inorganic metal oxide-based hetero-structured device, as Al/Al2O3/Nbx Oy /Au device. Reprinted from Ref. [52 ]. (c) Chemical diagram and schematic of polymer-based PVK-C60 memristive device. Reprinted from Ref. [58 ]. (d) Naturally extracted nanocellulose-based memristive device. Reprinted from Ref. [65 ]. (e) 2-dimensional (2D) shape of graphene. Reprinted from Ref. [71 ]. (f) 2D material MoS2, sandwiched between graphene electrode-based memristive device. Reprinted from Ref. [61 ]. (g) 3-terminal memristive device based on WSe2 2D material. Reprinted from Ref. [64 ]. (h) Schematic of PVPy-doped Au@Ag bimetal nanoparticle memristor storage. Reprinted from Ref [72 ]. (i) Sketch of an Ag/MAPbI3/FTO organic-inorganic hybrid perovskite artificial synapse device. Reprinted from Ref. [32 ], (j) Graphene oxide (GO) quantum dots (QDs) -based memristive storage device. Reprinted from Ref. [73 ], (k) InP/ZnS core-shell QDs-based memristive device, applied as an artificial synapse. Reprinted from Ref. [74 ].
![(Color online) Memristor and resistive switching characteristics. (a) Atomic force micrograph of 17 × 17 nano-cross bar array: the inset shows the schematic structure of the cross point. Reprinted from Ref. [76]. (b) Representation of biological synapse, including pre-/postsynaptic neurons and synaptic cleft. Reprinted from Ref. [73]. (c) Bipolar resistive switching characteristic with insets depicting the electroforming process and scanning electron microscope image of a 100 × 100 μm2 device. Reprinted from Ref. [77]. (d) Unipolar resistive switching characteristics. (e) Multi-level resistance states at different compliance currents. Reprinted from Ref. [78]. (f) The gradual switching performance of a Si memristor with an Ag–Ti alloy. Reprinted from Ref. [75]. (g) The complementary resistive switching behavior achieved by tailoring nanoplateau structures using solution-processed rutile TiO2 thin films. Reprinted from Ref. [30]. (h) Analog resistive characteristic induced by the structural engineering of a tantalum oxide-based memristor. Reprinted from Ref. [56]. (i) The threshold resistive switching I–V characteristics of N-GOQDs-based devices. The inset shows the controlled repetitive threshold switching I–V characteristics of the device under an Icc of 50 µA. Reprinted from Ref. [73]](/richHtml/jos/2021/42/1/013101/img_2.jpg)
Fig. 2. (Color online) Memristor and resistive switching characteristics. (a) Atomic force micrograph of 17 × 17 nano-cross bar array: the inset shows the schematic structure of the cross point. Reprinted from Ref. [76 ]. (b) Representation of biological synapse, including pre-/postsynaptic neurons and synaptic cleft. Reprinted from Ref. [73 ]. (c) Bipolar resistive switching characteristic with insets depicting the electroforming process and scanning electron microscope image of a 100 × 100 μ m2 device. Reprinted from Ref. [77 ]. (d) Unipolar resistive switching characteristics. (e) Multi-level resistance states at different compliance currents. Reprinted from Ref. [78 ]. (f) The gradual switching performance of a Si memristor with an Ag–Ti alloy. Reprinted from Ref. [75 ]. (g) The complementary resistive switching behavior achieved by tailoring nanoplateau structures using solution-processed rutile TiO2 thin films. Reprinted from Ref. [30 ]. (h) Analog resistive characteristic induced by the structural engineering of a tantalum oxide-based memristor. Reprinted from Ref. [56 ]. (i) The threshold resistive switching I–V characteristics of N-GOQDs-based devices. The inset shows the controlled repetitive threshold switching I–V characteristics of the device under an I cc of 50 µ A. Reprinted from Ref. [73 ]
![(Color online) Memristor synapse mimicking the representative synaptic functions of biological synapses for neuromorphic computing applications. (a) Synaptic potentiation and depression behavior of biological synapses obtained from a pair of glutamatergic neurons in hippocampal culture. (b) STDP behavior of biological synapse. Reprinted from Ref. [105]. (c) LTP/LTD characteristics exhibited by a memristor synapse. (d) STDP behavior, mimicked by a memristor-based artificial synapse, which follows the asymmetric Hebbian learning rule. Reprinted from Ref. [106].](/Images/icon/loading.gif)
Fig. 3. (Color online) Memristor synapse mimicking the representative synaptic functions of biological synapses for neuromorphic computing applications. (a) Synaptic potentiation and depression behavior of biological synapses obtained from a pair of glutamatergic neurons in hippocampal culture. (b) STDP behavior of biological synapse. Reprinted from Ref. [105 ]. (c) LTP/LTD characteristics exhibited by a memristor synapse. (d) STDP behavior, mimicked by a memristor-based artificial synapse, which follows the asymmetric Hebbian learning rule. Reprinted from Ref. [106 ].
![(Color online) Memristors with ternary - ABOx oxide storage. (a) TEM image of ALD Al2O3, HfO2, and HfAlOx based memory devices. (b) I–V characteristics of Al2O3, HfO2 and HfAlOx based memristors. (c) Switching mechanism of HfAlOx based memory device. Reprinted from Ref. [53]. (d) HZO thin film-based memory containing a network of grain boundaries, (e) Current-voltage hysteresis loops of the device. Reprinted from Ref. [115]. (f) STEM cross-sectional image of a 6 and 60 interface Gd0.1Ce0.9O2-δ/Er2O3 multilayer device. (g) I–V curve of the 60 interface memory device with a strain of –1.26%. Reprinted from Ref. [116]. (h) Hysteretic R–V characteristics of the memory device. (i) Device R–V memory state characteristics. Reprinted from Ref. [117].](/Images/icon/loading.gif)
Fig. 4. (Color online) Memristors with ternary - ABOx oxide storage. (a) TEM image of ALD Al2O3, HfO2, and HfAlOx based memory devices. (b) I–V characteristics of Al2O3, HfO2 and HfAlOx based memristors. (c) Switching mechanism of HfAlOx based memory device. Reprinted from Ref. [53 ]. (d) HZO thin film-based memory containing a network of grain boundaries, (e) Current-voltage hysteresis loops of the device. Reprinted from Ref. [115 ]. (f) STEM cross-sectional image of a 6 and 60 interface Gd0.1Ce0.9O2-δ/Er2O3 multilayer device. (g) I–V curve of the 60 interface memory device with a strain of –1.26%. Reprinted from Ref. [116 ]. (h) Hysteretic R–V characteristics of the memory device. (i) Device R–V memory state characteristics. Reprinted from Ref. [117 ].
![(Color online) Doping effects and modulation of memory oxide storage. (a) Resistive switching effect variations in HfO2–x oxygen vacancy modulated thin films. (b) Switching mechanism of HfO2–x oxygen vacancy modulated oxide storage. (c) Depth of resistance switching in HfO2–x oxygen vacancy modulated thin films. Reprinted from Ref. [41]. (d) Argon plasma pre-treated TaOx/IGZO-based memristive device. (e) Cumulative distribution of resistance of TaOx/IGZO-based memory device. Reprinted from Ref. [127]. (f) Schematic and fabrication process of metal nano-island embedded into HfO2 matrix, functioning as memristor storage. (g) I–V response of the device with b-PtD90 and m-PtD90 nano-islands embedded at the bottom electrode. Reprinted from Ref. [128]. (h) Si-doped Ta2O5–x memristor device displays an enlarged resistance ratio at RESET pulse operation. (i) Potential energy of ion hopping can be effectively modulated by, e.g. Si doping. Reprinted from Ref. [129]. (j) Ag cluster implantation could yield an analog-type memristor. Reprinted from Ref. [130]. (k) Zn doping into CeO2 oxide alters ion mobility in a CeO2 memristor storage oxide. Reprinted from Ref. [131].](/Images/icon/loading.gif)
Fig. 5. (Color online) Doping effects and modulation of memory oxide storage. (a) Resistive switching effect variations in HfO2–x oxygen vacancy modulated thin films. (b) Switching mechanism of HfO2–x oxygen vacancy modulated oxide storage. (c) Depth of resistance switching in HfO2–x oxygen vacancy modulated thin films. Reprinted from Ref. [41 ]. (d) Argon plasma pre-treated TaOx /IGZO-based memristive device. (e) Cumulative distribution of resistance of TaOx /IGZO-based memory device. Reprinted from Ref. [127 ]. (f) Schematic and fabrication process of metal nano-island embedded into HfO2 matrix, functioning as memristor storage. (g) I–V response of the device with b-PtD90 and m-PtD90 nano-islands embedded at the bottom electrode. Reprinted from Ref. [128 ]. (h) Si-doped Ta2O5–x memristor device displays an enlarged resistance ratio at RESET pulse operation. (i) Potential energy of ion hopping can be effectively modulated by, e.g. Si doping. Reprinted from Ref. [129 ]. (j) Ag cluster implantation could yield an analog-type memristor. Reprinted from Ref. [130 ]. (k) Zn doping into CeO2 oxide alters ion mobility in a CeO2 memristor storage oxide. Reprinted from Ref. [131 ].
![(Color online) Bilayer memristors with heterostructured and homostructured switching materials. (a) Cross-sectional TEM image of the IGZO/MnO heterostructured bilayer device. (b) Typical I–V characteristics of the heterostructured device, presenting repeatable multilevel bipolar switching. (c) The switching mechanism for volatile switching and multilevel bipolar switching. Reprinted from Ref. [37]. I–V characteristics of an Al2O3-based homostructured device, presenting multilevel unipolar switching characteristics during the (d) SET process and (e) RESET process. (f) Schematic representation of the switching mechanisms for multilevel unipolar switching behavior. Reprinted from Ref. [144]. (g) I–V characteristics of an HfO2/TiO2 bilayer device, exhibiting highly repeatable bipolar switching characteristics. (h) The endurance characteristics of the bilayer device, presenting excellent repeatability in HRS and LRS without any prominent degradation. (i) Schematic of the switching mechanism, presenting the formation and rupture of localized conductive filaments at the HfO2/TiO2 interface, which is responsible for the excellent reliability of the switching mechanism. Reprinted from Ref. [145].](/Images/icon/loading.gif)
Fig. 6. (Color online) Bilayer memristors with heterostructured and homostructured switching materials. (a) Cross-sectional TEM image of the IGZO/MnO heterostructured bilayer device. (b) Typical I–V characteristics of the heterostructured device, presenting repeatable multilevel bipolar switching. (c) The switching mechanism for volatile switching and multilevel bipolar switching. Reprinted from Ref. [37 ]. I–V characteristics of an Al2O3-based homostructured device, presenting multilevel unipolar switching characteristics during the (d) SET process and (e) RESET process. (f) Schematic representation of the switching mechanisms for multilevel unipolar switching behavior. Reprinted from Ref. [144 ]. (g) I–V characteristics of an HfO2/TiO2 bilayer device, exhibiting highly repeatable bipolar switching characteristics. (h) The endurance characteristics of the bilayer device, presenting excellent repeatability in HRS and LRS without any prominent degradation. (i) Schematic of the switching mechanism, presenting the formation and rupture of localized conductive filaments at the HfO2/TiO2 interface, which is responsible for the excellent reliability of the switching mechanism. Reprinted from Ref. [145 ].
![(Color online) Tuning switching behaviors by controlling the stacking sequence of the switching layers in bilayer memristors. Typical I–V characteristics of the single-layer (a) SnO2 and (b) IGZO devices, both presenting bipolar resistive switching. Typical I–V characteristics of bilayer-layer devices with (c) SnO2/IGZO stacking, exhibiting bipolar resistive switching, and (d) IGZO/SnO2 stacking, presenting unipolar switching characteristics. Schematic representations of the switching mechanisms during (e) bipolar switching in a SnO2/IGZO device and (f) unipolar switching in a reverse-stacked IGZO/SnO2 device. Reprinted from Ref. [80]. I–V characteristics indicating the coexistence of bipolar and unipolar switching in the single-layer (g) TaOx and (i) HfO2 device. (k) I–V characteristics demonstrating the existence of unipolar-only switching characteristics in a bilayer TaOx/HfO2 device. Reprinted from Ref. [149].](/Images/icon/loading.gif)
Fig. 7. (Color online) Tuning switching behaviors by controlling the stacking sequence of the switching layers in bilayer memristors. Typical I–V characteristics of the single-layer (a) SnO2 and (b) IGZO devices, both presenting bipolar resistive switching. Typical I–V characteristics of bilayer-layer devices with (c) SnO2/IGZO stacking, exhibiting bipolar resistive switching, and (d) IGZO/SnO2 stacking, presenting unipolar switching characteristics. Schematic representations of the switching mechanisms during (e) bipolar switching in a SnO2/IGZO device and (f) unipolar switching in a reverse-stacked IGZO/SnO2 device. Reprinted from Ref. [80 ]. I–V characteristics indicating the coexistence of bipolar and unipolar switching in the single-layer (g) TaOx and (i) HfO2 device. (k) I–V characteristics demonstrating the existence of unipolar-only switching characteristics in a bilayer TaOx /HfO2 device. Reprinted from Ref. [149 ].
![(Color online) Engineering of the switching modes of single-layer memristors using bilayers, and by controlling the composition of the component materials in the bilayer structures. (a) Schematic of a bilayer device structure, with a cross-sectional TEM image. I–V characteristics of devices fabricated using different oxygen partial pressures during TaOy deposition, exhibiting switching behaviors as (b) bipolar resistive switching for 3% oxygen partial pressure, (c) complementary resistive switching for 10% oxygen partial pressure, and (d) unipolar resistive switching for 15% oxygen partial pressure. Reprinted from Ref. [146]. (e) The abrupt digital switching characteristics in an HfO2 single-layer device. (f) The realization of gradual switching in a bilayer TaOx/HfO2 bilayer device. Reprinted from Ref. [150].](/Images/icon/loading.gif)
Fig. 8. (Color online) Engineering of the switching modes of single-layer memristors using bilayers, and by controlling the composition of the component materials in the bilayer structures. (a) Schematic of a bilayer device structure, with a cross-sectional TEM image. I–V characteristics of devices fabricated using different oxygen partial pressures during TaOy deposition, exhibiting switching behaviors as (b) bipolar resistive switching for 3% oxygen partial pressure, (c) complementary resistive switching for 10% oxygen partial pressure, and (d) unipolar resistive switching for 15% oxygen partial pressure. Reprinted from Ref. [146 ]. (e) The abrupt digital switching characteristics in an HfO2 single-layer device. (f) The realization of gradual switching in a bilayer TaOx /HfO2 bilayer device. Reprinted from Ref. [150 ].
![(Color online) Tunability of switching behaviors with bilayer and doped switching materials for improved emulation of synaptic functions. (a) Cross-sectional TEM image of a bilayer ZrO2/ZTO memristor device. The realization of a gradual multilevel switching in the bilayer device by controlling (b) compliance current during the SET process and (c) RESET-stop voltage during the RESET process. (d) Synaptic conductance modulation mimicking LTP and LTD behaviors. (e) Successful emulation of inter-spiking interval dependent PPF behavior, evaluated for various pulse intervals. (f) Experimental demonstration of STDP learning rule. Reprinted from Ref. [106]. (g) An illustration of spatial and temporal variations in the synaptic weight update process. Reprinted from Ref. [157]. (h) Schematic image of an HfO-based device, treated with Al-doping and post-deposition annealing methods. (i) Analog switching characteristics of a doped and annealed device. (j) Emulation of potentiation and depression behaviors with excellent linearity. (k) Simulation of STDP behavior. Reprinted from Ref. [158].](/Images/icon/loading.gif)
Fig. 9. (Color online) Tunability of switching behaviors with bilayer and doped switching materials for improved emulation of synaptic functions. (a) Cross-sectional TEM image of a bilayer ZrO2/ZTO memristor device. The realization of a gradual multilevel switching in the bilayer device by controlling (b) compliance current during the SET process and (c) RESET-stop voltage during the RESET process. (d) Synaptic conductance modulation mimicking LTP and LTD behaviors. (e) Successful emulation of inter-spiking interval dependent PPF behavior, evaluated for various pulse intervals. (f) Experimental demonstration of STDP learning rule. Reprinted from Ref. [106 ]. (g) An illustration of spatial and temporal variations in the synaptic weight update process. Reprinted from Ref. [157 ]. (h) Schematic image of an HfO-based device, treated with Al-doping and post-deposition annealing methods. (i) Analog switching characteristics of a doped and annealed device. (j) Emulation of potentiation and depression behaviors with excellent linearity. (k) Simulation of STDP behavior. Reprinted from Ref. [158 ].
![(Color online) Structural engineering of switching materials using rapid thermal annealing (RTA). Cross-sectional TEM images of the (a) as-deposited device without RTA and (b) RTA-processed device. Typical I–V characteristics of (c) non-RTA device with digital SET and analog RESET behaviors and (d) RTA-processed device with reliable bidirectional analog switching characteristics. (e) Repeatable potentiation and depression characteristics of the RTA-processed device. (f) SRDP behavior of the RTA-processed device, evaluated by the pulse trains with different pulse intervals. (g) The pulse amplitude-dependent electric response of the RTA-processed device with pulse trains having the same intervals but different heights. (h) Emulation of the Hermann Ebbinghaus forgetting curves. Reprinted from Ref. [56].](/Images/icon/loading.gif)
Fig. 10. (Color online) Structural engineering of switching materials using rapid thermal annealing (RTA). Cross-sectional TEM images of the (a) as-deposited device without RTA and (b) RTA-processed device. Typical I–V characteristics of (c) non-RTA device with digital SET and analog RESET behaviors and (d) RTA-processed device with reliable bidirectional analog switching characteristics. (e) Repeatable potentiation and depression characteristics of the RTA-processed device. (f) SRDP behavior of the RTA-processed device, evaluated by the pulse trains with different pulse intervals. (g) The pulse amplitude-dependent electric response of the RTA-processed device with pulse trains having the same intervals but different heights. (h) Emulation of the Hermann Ebbinghaus forgetting curves. Reprinted from Ref. [56 ].
![(Color online) Artificial synapse characteristics of a cone-shaped n-ZnO based memristor. (a) Schematic of fabricated cone-shaped n-ZnO based memory device. (b) TEM images of n-ZnO cone-shaped profile, equipped with EDS analysis, displaying stoichiometry of n-ZnO in separated regions, (c) low-current analog multi-level resistive switching I–V of the memristor, (d) multi-level resistance states retention characteristics of the memory device, (e) synapse device short-term and long-term memory characteristics after different number (#) of identical spikes applied, (f) synapse device frequency-related Schottky diode mechanism operation, (g) excitatory post-synaptic current (EPSC) change of the synapse device, depending on a variety of stimulation frequencies, and (h) learning-forgetting-re-learning behavior simulated in cone-shaped n-ZnO based memristor synapse. Reprinted from Ref. [55].](/Images/icon/loading.gif)
Fig. 11. (Color online) Artificial synapse characteristics of a cone-shaped n-ZnO based memristor. (a) Schematic of fabricated cone-shaped n-ZnO based memory device. (b) TEM images of n-ZnO cone-shaped profile, equipped with EDS analysis, displaying stoichiometry of n-ZnO in separated regions, (c) low-current analog multi-level resistive switching I–V of the memristor, (d) multi-level resistance states retention characteristics of the memory device, (e) synapse device short-term and long-term memory characteristics after different number (#) of identical spikes applied, (f) synapse device frequency-related Schottky diode mechanism operation, (g) excitatory post-synaptic current (EPSC) change of the synapse device, depending on a variety of stimulation frequencies, and (h) learning-forgetting-re-learning behavior simulated in cone-shaped n-ZnO based memristor synapse. Reprinted from Ref. [55 ].
![(Color online) Frequency–spike-rate dependent plasticity (SRDP) synapse characteristics of memristors. (a) plasticity modulation of synapse device by frequency in an Ag/PEDOT:PSS/Ta memristor. (b) High-frequency stimulation spiking increases current via Ag/PEDOT:PSS/Ta memristor. Reprinted from Ref. [166]. (c) Higher post-spiking firing rate increases the synaptic weight of an Ag/AgInSbTe/Ag memristor. (d) Lower spiking induces synapse depression, while higher spiking induces potentiation of the Ag/AgInSbTe/Ag synapse device. Reprinted from Ref. [167]. (e) Paired-pulse facilitation (PPF) characteristics of a Pd/WOx/W memristor. (f) frequency modulated current increase in the Pd/WOx/W synapse device. Reprinted from Ref. [92]. (g) Second-order memristor operation schematic of a Pd/Ta2O5–x/TaOy/Pd memristor. (h) Spiking frequency dependence vs. spiking number and conductance change in the Pd/Ta2O5–x/TaOy/Pd synapse device. Reprinted from Ref. [168]. (i) Orthorhombic phase in HfOy/HfOx oxide storage, affecting the conductance state of the memristor. (j) Plasticity of the synapse, regulated by spiking frequency. Reprinted from Ref. [139]. (k) Memristor top electrode engineering with Pt-Al alloy co-sputtering. (l) Dependence on Pt concentration of Pt-Al alloy, resulting in variations in the synapse spiking frequency characteristics of the device. Reprinted from Ref. [169].](/Images/icon/loading.gif)
Fig. 12. (Color online) Frequency–spike-rate dependent plasticity (SRDP) synapse characteristics of memristors. (a) plasticity modulation of synapse device by frequency in an Ag/PEDOT:PSS/Ta memristor. (b) High-frequency stimulation spiking increases current via Ag/PEDOT:PSS/Ta memristor. Reprinted from Ref. [166 ]. (c) Higher post-spiking firing rate increases the synaptic weight of an Ag/AgInSbTe/Ag memristor. (d) Lower spiking induces synapse depression, while higher spiking induces potentiation of the Ag/AgInSbTe/Ag synapse device. Reprinted from Ref. [167 ]. (e) Paired-pulse facilitation (PPF) characteristics of a Pd/WOx /W memristor. (f) frequency modulated current increase in the Pd/WOx /W synapse device. Reprinted from Ref. [92 ]. (g) Second-order memristor operation schematic of a Pd/Ta2O5–x /TaOy /Pd memristor. (h) Spiking frequency dependence vs. spiking number and conductance change in the Pd/Ta2O5–x /TaOy /Pd synapse device. Reprinted from Ref. [168 ]. (i) Orthorhombic phase in HfOy/HfOx oxide storage, affecting the conductance state of the memristor. (j) Plasticity of the synapse, regulated by spiking frequency. Reprinted from Ref. [139 ]. (k) Memristor top electrode engineering with Pt-Al alloy co-sputtering. (l) Dependence on Pt concentration of Pt-Al alloy, resulting in variations in the synapse spiking frequency characteristics of the device. Reprinted from Ref. [169 ].
![(Color online) Synaptic crossbar arrays with volatile threshold switching for the emulation of synaptic plasticity. (a) SEM image of a fabricated crossbar array, with a magnified image of a single cross-point memristor cell. (b) Cross-sectional TEM image of a single memristor cell with structure Ag/HfO2/Pt. The FFT patterns indicate the morphology of the corresponding thin films. (c) Schematics of a biological synapse and a fabricated electronic synapse, presenting the correlation between the two. (d) Typical I–V characteristics of the volatile threshold resistive switching behavior of the device. The inset shows nonvolatile switching behavior at a higher compliance current. (e) Cross-sectional TEM image of the device, confirming Ag conductive filament formation when switched to LRS with a higher compliance current. (f) Delay, SET, and self-relaxation characteristics of the device. The inset shows the exponential decay fitting of the current relaxation. (g) Experimental demonstration of STP and LTP behaviors with a transition from STP to LTP. (h) STDP characteristics of the device with a pulse scheme of pre-synaptic and post-synaptic spiking. Reprinted from Ref. [49].](/Images/icon/loading.gif)
Fig. 13. (Color online) Synaptic crossbar arrays with volatile threshold switching for the emulation of synaptic plasticity. (a) SEM image of a fabricated crossbar array, with a magnified image of a single cross-point memristor cell. (b) Cross-sectional TEM image of a single memristor cell with structure Ag/HfO2/Pt. The FFT patterns indicate the morphology of the corresponding thin films. (c) Schematics of a biological synapse and a fabricated electronic synapse, presenting the correlation between the two. (d) Typical I–V characteristics of the volatile threshold resistive switching behavior of the device. The inset shows nonvolatile switching behavior at a higher compliance current. (e) Cross-sectional TEM image of the device, confirming Ag conductive filament formation when switched to LRS with a higher compliance current. (f) Delay, SET, and self-relaxation characteristics of the device. The inset shows the exponential decay fitting of the current relaxation. (g) Experimental demonstration of STP and LTP behaviors with a transition from STP to LTP. (h) STDP characteristics of the device with a pulse scheme of pre-synaptic and post-synaptic spiking. Reprinted from Ref. [49 ].
![(Color online) Synaptic memristor based on nitrogen-doped graphene oxide (N-GOQDs). (a) TEM cross-section image of N-GOQDs-based device, in an Ag/N-GOQDs/Pt structure. (b) Schematic of the fabricated device, depicting Ag ion migration via various functional groups in the N-GOQDs matrix. (c) I–V characteristics of threshold resistive switching (TS) behavior of the device; inset shows continuous dc cycling (#50) of the device. (d) Delay-relax characteristics of the N-GOQDs-based memristor; inset shows the time-rated spontaneous dissolution of Ag filament. (e) AC endurance ‘firing’ characteristics of the device,up to 1.2 × 104 cycles. (f) Paired-pulse facilitation (PPF) synaptic characteristics of the device. (g) STP and LTP memory states emulated in N-GOQDs-based synapse device. (h) Spike-timing-dependent plasticity (STDP) characteristics of the device, obtained from bipolar switching in higher current compliance, as shown in the inset, respectively. Reprinted from Ref. [73].](/Images/icon/loading.gif)
Fig. 14. (Color online) Synaptic memristor based on nitrogen-doped graphene oxide (N-GOQDs). (a) TEM cross-section image of N-GOQDs-based device, in an Ag/N-GOQDs/Pt structure. (b) Schematic of the fabricated device, depicting Ag ion migration via various functional groups in the N-GOQDs matrix. (c) I–V characteristics of threshold resistive switching (TS) behavior of the device; inset shows continuous dc cycling (#50) of the device. (d) Delay-relax characteristics of the N-GOQDs-based memristor; inset shows the time-rated spontaneous dissolution of Ag filament. (e) AC endurance ‘firing’ characteristics of the device,up to 1.2 × 104 cycles. (f) Paired-pulse facilitation (PPF) synaptic characteristics of the device. (g) STP and LTP memory states emulated in N-GOQDs-based synapse device. (h) Spike-timing-dependent plasticity (STDP) characteristics of the device, obtained from bipolar switching in higher current compliance, as shown in the inset, respectively. Reprinted from Ref. [73 ].
![(Color online) Organic storage memristor and leaky-integrate and fire (LIF) characteristics. (a) PVK-C60-based memory, switching mechanism of RS behavior, and corresponding J–V of bipolar resistive switching characteristics of an ITO/PVK-C60/Al device. Reprinted from Ref. [58]. (b) Block co-polymer (PVPCz59) based memristor, displaying unipolar resistive switching in I-V characteristics. Reprinted from Ref. [179]. (c) Albumen-based memristor, prepared by heat-denaturation of proteins, showing the reliable RS I–V characteristics of the device. Reprinted from Ref. [180]. (d) Memristor based on soft-wood nanocellulose, indicating repeatable I–V of RS behavior of the device via C-AFM measurement. Reprinted from Ref. [65]. (e) Biological representation of leaky-integrate and fire (LIF) process with synapses and neurons; adapted LIF circuit with threshold resistive switching device installation, analogous to biology; typical Vin–Vout characteristics of the LIF circuit with TS memory device. Reprinted from Ref. [74].](/Images/icon/loading.gif)
Fig. 15. (Color online) Organic storage memristor and leaky-integrate and fire (LIF) characteristics. (a) PVK-C60-based memory, switching mechanism of RS behavior, and corresponding J–V of bipolar resistive switching characteristics of an ITO/PVK-C60/Al device. Reprinted from Ref. [58 ]. (b) Block co-polymer (PVPCz59) based memristor, displaying unipolar resistive switching in I-V characteristics. Reprinted from Ref. [179 ]. (c) Albumen-based memristor, prepared by heat-denaturation of proteins, showing the reliable RS I–V characteristics of the device. Reprinted from Ref. [180 ]. (d) Memristor based on soft-wood nanocellulose, indicating repeatable I–V of RS behavior of the device via C-AFM measurement. Reprinted from Ref. [65 ]. (e) Biological representation of leaky-integrate and fire (LIF) process with synapses and neurons; adapted LIF circuit with threshold resistive switching device installation, analogous to biology; typical V in–V out characteristics of the LIF circuit with TS memory device. Reprinted from Ref. [74 ].
![(Color online) Memristor advances in 2D material devices. (a) Memristor device schematic with 2D (PEA)2PbBr4 perovskite single crystal-based memory storage and TEM cross-section image of the device. (b) Experimentally measured low-current STP & LTP characteristics of memristor synapse device. Reprinted from Ref. [ 60]. (c) Schematic of MoOx-based optical RRAM memristor. (d) Sketch of the human visual system. (e) Illustration of the crossbar (6 × 7) with optical memristor synapse devices, as an artificial neuromorphic system for image pre-processing and image recognition, respectively. Reprinted from Ref. [62].](/Images/icon/loading.gif)
Fig. 16. (Color online) Memristor advances in 2D material devices. (a) Memristor device schematic with 2D (PEA)2PbBr4 perovskite single crystal-based memory storage and TEM cross-section image of the device. (b) Experimentally measured low-current STP & LTP characteristics of memristor synapse device. Reprinted from Ref. [ 60 ]. (c) Schematic of MoOx -based optical RRAM memristor. (d) Sketch of the human visual system. (e) Illustration of the crossbar (6 × 7) with optical memristor synapse devices, as an artificial neuromorphic system for image pre-processing and image recognition, respectively. Reprinted from Ref. [62 ].
![(Color online) Advances in memristor devices. (a) Schematic of hetero-synaptic plasticity synapse device, influenced by neuro-modulatory axon. (b) Hetero-synaptic memristor device. (c) Pre-synaptic pulse operation, influenced by modulatory bias. Reprinted from Ref. [182]. (d) Multi-terminal synapse device, consisting of multiple memristors, exhibiting cooperative behavior. Reprinted from Ref. [183]. (e) Artificial afferent nerve designed using pressure sensors, ring oscillators and synaptic memristive transistor. Reprinted from Ref. [184].](/Images/icon/loading.gif)
Fig. 17. (Color online) Advances in memristor devices. (a) Schematic of hetero-synaptic plasticity synapse device, influenced by neuro-modulatory axon. (b) Hetero-synaptic memristor device. (c) Pre-synaptic pulse operation, influenced by modulatory bias. Reprinted from Ref. [182 ]. (d) Multi-terminal synapse device, consisting of multiple memristors, exhibiting cooperative behavior. Reprinted from Ref. [183 ]. (e) Artificial afferent nerve designed using pressure sensors, ring oscillators and synaptic memristive transistor. Reprinted from Ref. [184 ].
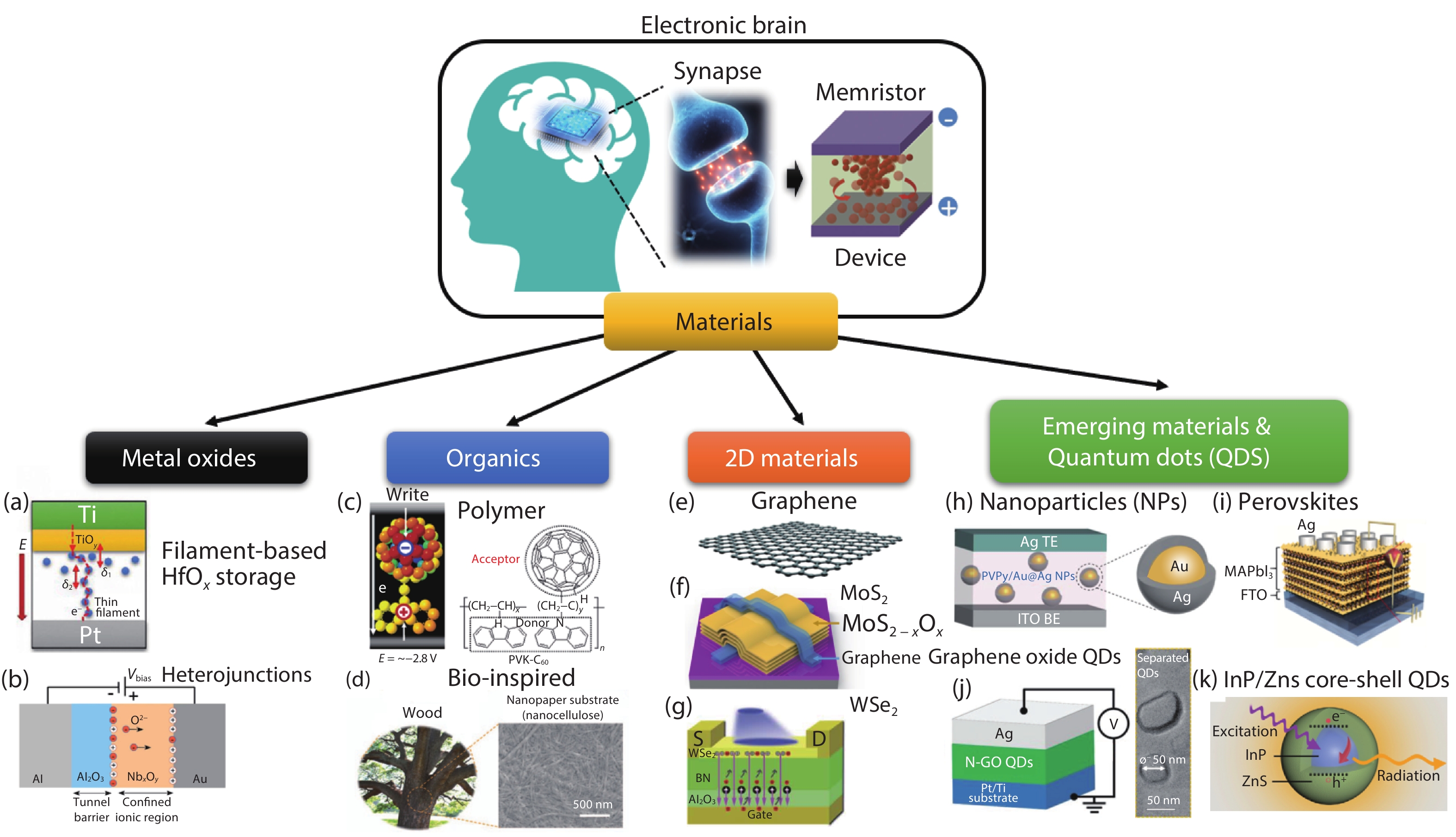
Set citation alerts for the article
Please enter your email address