Author Affiliations
1Fang Lu Mesoscopic Optics and Quantum Electronics Laboratory, University of California, Los Angeles, California 90095, USA2Current address: Division of Physical Metrology, Korea Research Institute of Standards and Science (KRISS), Daejeon 34113, Republic of Korea3Current address: CACI-LGS Labs, Florham Park, New Jersey 07932, USA4Department of Mechanical Engineering, Korea Advanced Institute of Science and Technology (KAIST), Daejeon 34141, Republic of Korea5OEwaves Inc., Pasadena, California 91107, USA6Jet Propulsion Laboratory, California Institute of Technology, Pasadena, California 91109, USA7e-mail: ysj@kriss.re.krshow less
Fig. 1. Conceptual image of sub-femtometer/Hz1/2 scale displacement measurement. (a) Architecture of the sub-femtometer/Hz1/2 displacement measurement. A laser stabilized to reference cavity with 10−15 fractional stability at 1 s is used as a frequency reference, while an SSB modulator locks the laser frequency to the WGM microcavity resonance mode. (b) Frequency-domain configuration of sub-femtometer/Hz1/2 displacement measurement. The reference laser frequency (fref) is shifted with SSB modulator by fSSB to lock to the microcavity resonance. Since the reference laser has 105 higher fractional stability than the microcavity, a fluctuation of frequency shift (fSSB) reflects the fluctuation of the microcavity.
Fig. 2. Sub-femtometer/Hz1/2 displacement measurement setup. (a) The FP cavity stabilized laser provides the frequency reference point. The PDH scheme generates the error signal to synchronize the reference laser frequency (fref) with the MgF2 WGM microcavity resonance mode (fWGM) using the SSB modulator. Fluctuation of the WGM microcavity is recorded by the FFT spectrum analyzer and frequency counter. The inset shows the PDH error signal near the MgF2 microcavity resonance. SSB modulator, single-sideband modulator; EDFA, erbium-doped fiber amplifier; FBG, fiber Bragg grating; EOM, electro-optic modulator; WGM microcavity, whispering-gallery-mode microcavity; PD, photodetector; RF synthesizer, radio-frequency synthesizer; RF LPF, radio-frequency low-pass filter; PI servo, proportional-integral servo controller. (b) Upper figure shows vacuum chamber system. Lower figure shows the optical ring-down measurement of the MgF2 microcavity.
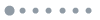
Fig. 3. Frequency noise and displacement on the MgF2 WGM microcavity. The power spectral density of frequency noise and displacement of the MgF2 WGM microcavity measured by the frequency shift (fSSB) for synchronization between the cavity stabilized laser and the MgF2 WGM microcavity. The left y axis shows the frequency noise in Hz/Hz1/2, and the right y axis shows the equivalent displacement in m/Hz1/2 units. Displacement of 1 fm/Hz1/2 corresponds to frequency noise of 16.7 Hz/Hz1/2. Frequency noises analyzed from the control signal (blue color) with frequency synchronization and PDH error signal (yellow color) without frequency synchronization are illustrated for comparison. Pink color denotes the frequency noise without vacuum, and the orange line denotes the combined thermal noise predictions from our theoretical model. PDH error signal after frequency synchronization (olive color) and noise floor of PDH error signal (gray color) are also illustrated.
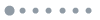
Fig. 4. Real-time trace of frequency shift and displacement on MgF2 WGM microcavity. (a) Example time trace of the MgF2 WGM microcavity resonance frequency shift during 300 s. Equivalent displacement on the MgF2 WGM microcavity is shown in right y axis. Inset is a magnified view from 20 s to 60 s. (b) Long-term time trace of the MgF2 WGM microcavity resonance frequency shift with extended locking range of 30 MHz during 4500 s. Inset is a magnified view from 3380 s to 3420 s. (c) Stability of the MgF2 microcavity resonance frequency. Equivalent fractional stability and displacement are plotted in the left and right y axis, respectively. The frequency stability estimated from frequency noise as shown in Fig. 3 for the short-term time (10−5 to 4.6 s) is plotted in blue. The frequency stability from the frequency counter for the long-term time scale (10−1 to 464 s) is plotted in green and yellow. The results are well matched in overlapped region of 10−1 to 4.6 s. The olive line is the estimated relative fractional stability between the frequency-shifted laser and MgF2 microcavity, bounded by our measurement repeatability.
Fig. 5. Real-time measurement of the response dynamics of the MgF2 WGM microcavity under thermal modulations. (a) Time trace of dynamic motion of the MgF2 WGM microcavity under thermal modulation with 0.1 to 2 Hz modulation frequency. (b) Amplitude modulation of resonance frequency of the MgF2 microcavity in relation to modulation frequency while modulation amplitude is fixed to 0.5 mA. Inset is its log-scale plot.
Fig. 6. Conceptual schematic of resonance tracking-based subfemtometer motional displacement measurements in linear Fabry–Perot cavities and interferometry. The red line and blue line correspond to the optical and electrical lines, respectively. The right mirror of the ultrahigh-Q FP cavity is mounted to the target.