Abstract
Virus is a kind of microorganism and possesses simple structure and contains one nucleic acid, which must be replicated using the host cell system. It causes large-scale infectious diseases and poses serious threats to the health, social well-being, and economic conditions of millions of people worldwide. Therefore, there is an urgent need to develop novel strategies for accurate diagnosis of virus infection to prevent disease transmission. Quantum dots (QDs) are typical fluorescence nanomaterials with high quantum yield, broad absorbance range, narrow and size-dependent emission, and good stability. QDs-based nanotechnology has been found to be effective method with rapid response, easy operation, high sensitivity, and good specificity, and has been widely applied for the detection of different viruses. However, until now, no systematic and critical review has been published on this important research area. Hence, in this review, we aim to provide a comprehensive coverage of various QDs-based virus detection methods. The fundamental investigations have been reviewed, including information related to the synthesis and biofunctionalization of QDs, QDs-based viral nucleic acid detection strategies, and QDs-based immunoassays. The challenges and perspectives regarding the potential application of QDs for virus detection is also discussed.Virus is a kind of microorganism and possesses simple structure and contains one nucleic acid, which must be replicated using the host cell system. It causes large-scale infectious diseases and poses serious threats to the health, social well-being, and economic conditions of millions of people worldwide. Therefore, there is an urgent need to develop novel strategies for accurate diagnosis of virus infection to prevent disease transmission. Quantum dots (QDs) are typical fluorescence nanomaterials with high quantum yield, broad absorbance range, narrow and size-dependent emission, and good stability. QDs-based nanotechnology has been found to be effective method with rapid response, easy operation, high sensitivity, and good specificity, and has been widely applied for the detection of different viruses. However, until now, no systematic and critical review has been published on this important research area. Hence, in this review, we aim to provide a comprehensive coverage of various QDs-based virus detection methods. The fundamental investigations have been reviewed, including information related to the synthesis and biofunctionalization of QDs, QDs-based viral nucleic acid detection strategies, and QDs-based immunoassays. The challenges and perspectives regarding the potential application of QDs for virus detection is also discussed.1. Introduction
Viruses are infectious entities, in which one kind of nucleic acid, either DNA or RNA, is wrapped in a protein shell with a diameter ranging from 16 nm to over 300 nm[1]. The nucleic acids are sometimes also encapsulated in a lipid membrane in some specific species. Since viruses do not contain ribosomes or any other cellular organelles, their only means of reproduction is to infect the living cells and replicate by using the ribosomes of host cells[2]. Emerging and reemerging viral infections can cause severe respiratory tract, intestinal tract, skin, single organ, multi-organ, nervous system, and hematological diseases, and adversely affect human the health[3-6]. In addition, numerous outbreaks of large-scale infection have occurred worldwide, especially the current outbreak of novel coronavirus disease 2019 (COVID-19)[7]. Due to the severity and damage caused by such infections, rapid, accurate, available, user-friendly, and low-cost detection methods for the detection of viruses are necessary[8,9]. The existing methods, such as virus culture, polymerase chain reaction (PCR)[10], enzyme linked immunosorbent assay (ELISA)[11] and other serological methods[12], are widely used in clinical practice. These methods are extremely sensitive and specific, however, they require expensive equipment and professional operators and suffer from major drawbacks of being time consuming and labor intensive[13,14].
Nanobiosensor is a kind of novel biosensor, which primarily depends on the specific recognition and interaction of nanomaterials with the molecular substances, such as enzymes, antigens, antibodies, and DNA, to transduce as optical and electrochemical signals[15]. Moreover, due to the high signals and large surface volume ratios of nanomaterials, nanobiosensors can determine extremely low analyte concentrations in a variety of biological samples with high accuracy, sensitivity, and specificity[9,16]. Interestingly, various kinds of nanomaterials, such as gold nanoparticles (Au NPs)[17], carbon nanotubes (CNTs)[18], magnetic nanoparticles[19] and quantum dots (QDs)[20] can be used for nanobiosensors. Among them, QDs are one of the best characterized nanomaterials with versatile optical and electrical properties, which can function as semiconductor nanocrystals and mainly made up of group II–VI, II–V or IV–VI[21]. Compared with organic fluorescent dyes, the QDs have unique properties such as broad excitation range, symmetric photoluminescence, multiplex staining, long fluorescent lifetime, and high resistance to photobleaching[22,23]. The main synthesis methods for QDs include organometallic synthesis and aqueous synthesis, and the aqueous QDs have been found to be more suitable for bioapplication[24]. In addition, the biofunctionalization of QDs is necessary, which can attach different biomolecules (oligonucleotides, proteins, peptides, and small molecules) onto the surface of QDs by various interactions (electrostatic interaction, covalently coupling, and metal-ligand interaction).
Recently, QDs have been actively exploited for virus detection by integrating novel analytical methods (e.g., fluorescence resonance energy transfer, aggregation induced quenching, magnetic separation, and enzyme-linked immunosorbent assay) with the functional materials (e.g., gold nanoparticle, graphene oxide, magnetic bead, and SiO2 microsphere)[25-28]. However, there are limited reports that have extensively reviewed strategies for QDs-based virus detection. Herein, we review the recent advances in QDs-based biosensors for viral nucleic acid, antibody, and antigen detection (Fig. 1). First, the synthesis and biofunctionalization of QDs was described. Then, we have mainly focused on viral nucleic acid detection, including nucleic acid hybridization, nucleic acid amplification, and probe signal enhancement. Later, we have introduced the strategies for viral antibody and antigen detection, namely, QDs-based immunoassays, including heterogeneous immunoassays (enzyme-linked immunosorbent assay, magnetic bead-based immunoassay, and lateral-flow immunochromatographic assay) and homogeneous immunoassays (fluorescence resonance energy transfer-based immunoassays). Finally, we have discussed the various challenges and future direction of QDs-based virus detection.
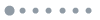
Figure 1.(Color online) Summary of potential applications of semiconductor quantum dots for virus detection.
2. Synthesis and biofunctionalization of QDs
QDs are typical zero-dimensional nanomaterials, including II–VI, III–V, or IV–VI nanomaterials[29]. However, compared with the traditional fluorescence dye and fluorescence proteins, QDs exhibit excellent optical properties, such as broad absorption spectrum, narrow and adjustable emission, high quantum yield, good photostability, and easy biological functionalization[30,31]. The methods used for synthesis of QDs include organometallic synthesis[32] and aqueous synthesis[33]. The QDs obtained by organometallic synthesis have uniform particle size, high quantum yield and good stability, but have been found to be highly toxic. The aqueous synthesis method is simple, green, and easy to surface functionalization, which can be directly used in various biological systems. The biological functionalization of QDs involves use of proteins, nucleic acids, or small biomolecules to modify the surface of nanoparticles, which has a wide range of applications in biosensors and bioimaging[34]. The major methods include electrostatic interaction, covalently coupling, and metal-ligand interaction.
2.1. Synthesis of QDs
2.1.1. Organometallic synthesis of QDs
Organometallic synthesis is conducted to obtain QDs by using hot injection or heating-up methods to facilitate the nucleation and growth of the precursors in organic solvents[32]. The hot injection method involves rapid addition of the precursor to the hot organic solvent for nucleation, and the precursor is continuously added to promote the growth[35]. The heating-up method is used to make the precursor locally supersaturated to nucleate and grow significantly by heating[36]. Water-soluble modification is necessary for the bio-application of organometallic QDs, which includes the ligand exchange[37], hydrophobic coating[38], and silanation[39]. Ligand exchange refers to one end of the hydrophilic molecule which contains sulfhydryl, pyridine, imidazole, or other structures, can replace the original ligand on the surface of QDs, and the hydrophilic groups at the other end to maintains its solubility in water[40]. The dissociation and binding of the ligands is a dynamic and reversible process, which can easily lead to the aggregation of QDs and the reduction of fluorescence intensity. Hydrophobic coating indicates that QD is coated by amphiphilic polymer, such as polymaleic anhydride, polyacrylamide, or polyethylene glycol, and the hydrophobic chain is embedded in the hydrophobic ligand on QD surface, with the hydrophilic end facing outward to achieve water solubility[38]. The method has good photostability, but the size of particles after coating is relatively large. Silanization can form the silica shell on the QD surface to cause it to stably disperse in water. Silica is non-toxic substance, has good biocompatibility and can significantly inhibit QDs photodegradation[41]. Silanization uses non-toxic silica, which has good biocompatibility, but has not been widely used due to its complex operation.
2.1.2. Aqueous synthesis of QDs
The aqueous synthesis of QDs mainly includes microwave[42], reflux[43], and hydrothermal methods[33], among which hydrothermal method is the most widely used. In the hydrothermal method, thiol-phosphorus precursors and metal precursors are prepared, respectively, and then mixed it to form QDs crystal nuclei, which are then transferred into Teflon-lined stainless-steel autoclave to further grow into QDs[44,45]. Thiol-phosphorus precursor is usually obtained by the reaction of Te/Se and NaBH4 under anaerobic conditions, and its product can be easily oxidized, so the transfer process requires rapid and skilled operation. Zhanget al. developed a one-pot hydrothermal method to prepare QDs using Na2TeO3 as Te source to simplify the process (Fig. 2(a))[46]. In this method, Te2− was obtained by reacting Na2TeO3 with NaBH4, which could directly interact with the metal precursor and did not involve the transfer of Te precursor. Surface modification of QDs can affect the particle size, shape, luminescence efficiency, cytotoxicity, cell penetration, and non-specific adsorption of QDs[47]. Thiols are the most commonly used surface modified ligands, which can interact strongly with the metal ions and stabilize QDs. QDs modified by monomercaptan ligands can exhibit good luminescence efficiency, but poor photostability, easy aggregation, and narrow pH range. Multimercaptan ligands have stronger interaction with the metal ions and can be used to modify QDs to obtain better stability. Maoet al. developed innovative methods for preparing the stable aqueous QDs using dithiol as the surface ligand, which could markedly improve fluorescence quantum yield and stability[48-52]. Zhanet al. used amino group and tetramercaptan as potential ligands for QDs synthesis, which can stably exist in high concentration of the salt, cell culture medium, or glutathione solution, and possess stable fluorescence properties in the pH range of 2–14 (Fig. 2(b))[53]. In addition, other ligands, such as imidazole and adenosine, can also stabilize QDs, but due to their poor interactions with metal ions, co-stabilizers are required. Wanget al. designed and synthesized a ligand containing both imidazole and dithiol, which exhibited the advantages of strong interaction between dithiol and metal ions and anti-oxidation of imidazole (Fig. 2(c))[37].
![(Color online) The different QDs based on types of ligands used for synthesis. (a) One-step preparation of DNA-QDs using Na2TeO3 as the tellurium source and N-acetyl-L-cysteine as the surface ligand[46]. (b) Amino group and tetramercaptan as the ligands for QDs synthesis using photoligation strategy[53]. (c) Imidazole and dithiol co-stabilized QDs to maintain strong interaction between dithiol and the metal ions and cause anti-oxidation of imidazole[37]. Modified with permission from (a) Ref. [46] Copyright 2014 American Chemical Society, (b) Ref. [53] Copyright 2013 American Chemical Society and (c) Ref. [37] Copyright 2015 American Chemical Society.](/Images/icon/loading.gif)
Figure 2.(Color online) The different QDs based on types of ligands used for synthesis. (a) One-step preparation of DNA-QDs using Na2TeO3 as the tellurium source and N-acetyl-L-cysteine as the surface ligand[46]. (b) Amino group and tetramercaptan as the ligands for QDs synthesis using photoligation strategy[53]. (c) Imidazole and dithiol co-stabilized QDs to maintain strong interaction between dithiol and the metal ions and cause anti-oxidation of imidazole[37]. Modified with permission from (a) Ref. [46] Copyright 2014 American Chemical Society, (b) Ref. [53] Copyright 2013 American Chemical Society and (c) Ref. [37] Copyright 2015 American Chemical Society.
2.2. Biofunctionalization of QDs
2.2.1. Electrostatic interaction
The combination of biomolecules and QDs via electrostatic adsorption is one of the simplest methods employed for biofunctionalization of QDs. Bhanget al. prepared HA-QDs by using negatively charged hyaluronic acid (HA) and positively charged QDs to detect hyaluronidase (Fig. 3(a))[54]. Although electrostatic adsorption is a simple and effective technique, it is difficult to avoid non-specific adsorption. Moreover, some biomolecules, such as protamine and sodium heparin, could easily cause aggregation and self-quenching of QDs.
![(Color online) Biofunctionalization of QDs. (a) The synthesis of hyaluronic acid functionalized QDs through electrostatic interaction[54]. (b) The preparation of azide-DNA functionalized QDs for GOx labeling through copper-free catalytic click reaction for the blood glucose detection[62]. (c) The design of two-dimensional QD molecular beacons through the coordination interaction of Cd2+ and dithiol[65]. Modified with permission from (a) Ref. [54] Copyright 2009 American Chemical Society, (b) Ref. [60] Copyright 2021 American Chemical Society and (c) Ref. [62] Copyright The Royal Society of Chemistry 2015.](/Images/icon/loading.gif)
Figure 3.(Color online) Biofunctionalization of QDs. (a) The synthesis of hyaluronic acid functionalized QDs through electrostatic interaction[54]. (b) The preparation of azide-DNA functionalized QDs for GOx labeling through copper-free catalytic click reaction for the blood glucose detection[62]. (c) The design of two-dimensional QD molecular beacons through the coordination interaction of Cd2+ and dithiol[65]. Modified with permission from (a) Ref. [54] Copyright 2009 American Chemical Society, (b) Ref. [60] Copyright 2021 American Chemical Society and (c) Ref. [62] Copyright The Royal Society of Chemistry 2015.
2.2.2. Covalently coupling
Covalent coupling is the most common method employed for biofunctionalization of QDs, mainly including the reaction of amino and carboxyl, hydrazine, and aldehyde, phenylboric acid and diol, and maleimide and sulfhydryl group[55-57]. Carbonized diimide chemical method has been used to activate the carboxyl group to obtain amide and promote the reaction between amino and amide to form ester[58]. Nie and Chanet al. covalently coupled thioglycolic acid stabilized CdSe/ZnS QDs with transferrin by using carbonized diimide chemical method to obtain transferrin-QDs[59,60]. Transferrin-QDs can specifically recognize the different surface receptors and can effectively enter into cervical cancer (HeLa) cells, which were used for cell imaging for the first time. Generally, antibodies contain the sulfhydryl groups, which can often react with maleimide modified QDs to quickly facilitate antibody labeling[55]. Multicolor QDs can be used to label the different antibodies to detect multiple antigens[55]. Although the conjugation between QDs and biomolecules is very stable, these coupling reactions can easily lead to the reduction of luminescence efficiency and stability of QDs. Recently, a novel covalent coupling method, click reaction, has attracted significant attention due to its mild reaction conditions, high coupling efficiency, and minimal cross coupling tendency. Click reaction usually requires copper ions as a catalyst, but it can effectively quench the QDs fluorescence and has cytotoxicity[61]. Maoet al. developed azide-DNA functionalized QDs for GOx labeling by copper-free catalytic click reaction to produce GOx-QDs, which could be employed for the rapid blood glucose detection with high sensitivity (Fig. 3(b))[62].
2.2.3. Metal-ligand interaction
Inspired by the biomineralization reaction between the metal ions and histidine-rich proteins, Clappet al. modified CdSe/ZnS QDs with histidine-containing biomolecules, which showed strong binding force and fast binding speed[63]. Because histidine is easy to fuse with different proteins, it is suitable for establishing the connection between QDs and proteins. Metal ions also have strong binding capacity with sulfhydryl or imidazole and are commonly used in biofunctionalized QDs[64]. In another study, Wu et al. synthesized a sulfhydryl modified DNA and prepared a two-dimensional QD-beacon through the combination of sulfhydryl and metal ions (Fig. 3(c))[65]. The BHQ3 and chlorin e6 were modified in DNA, which were used for imaging and photodynamic therapy, respectively. However, without directly targeting the microRNA, QDs fluorescence was quenched by BHQ3 and the photodynamic therapy was inhibited. In the present of the target microRNA, QDs fluorescence was recovered and the photodynamic therapy was inactivated. Maet al. designed a BHQ and phosphorothioate co-modified DNA to prepare QD nano-beacons with controllable valence for single RNA imaging and virus dynamic tracking in the live cells[66].
3. Virus detection based on QDs
Due to high quantum yield, photobleaching resistance, and high signal-to-noise ratio, QDs have displayed good application potential for the early diagnosis of viruses and the study of infection mechanisms in recent years. A number of QD-based methods have been developed for viral gene detection and protein immunoassay. The genetic material consisting of the nucleic acid plays a significant role in the virus replication, proliferation, heredity, and evolution, and is the main biomarker for the diagnosis of virus infection[67]. The principle of nucleic acid detection is based on nucleic acid hybridization with high specificity. A variety of DNA functionalized QDs probes have been constructed for viral nucleic acid detection, and the detection sensitivity was further improved through nucleic acid amplification and signal enhancement[22,68-70]. Immunoassay depends primarily on the specificity of antibodies and antigens binding to achieve the diagnosis of virus infection. However, compared with nucleic acid detection, the immunoassay is simple and convenient, does not require expensive instruments as well as the technical personnel, and is suitable for rapid virus screening. Immunoassays can be mainly divided into homogeneous and heterogeneous assays. Heterogeneous system can remove the free components from target analyte through washing step, which is an immensely popular analytical method with high sensitivity and versatility. On the contrary, homogeneous system has no solid support and does not require washing and separation process. It is suitable for the rapid detection and can improve sensitivity through signal amplification.
3.1. Molecule biology detection for virus infection diagnosis
3.1.1. Hybridization probe
The most common method for nucleic acid detection is to recover the fluorescence signals through promoting the hybridization of target nucleic acid and DNA probes, such as molecular beacon and DNA functionalized QD. QD hybridization probe is constructed by conjugation of the quencher with QD, and four conjugation methods have been identified, including electrostatic interaction, π–π stacking interaction, covalently coupling, and metal-ligand interaction. Jianget al. prepared positively charged carbon QDs and combined them with Dabcyl-modified DNA through electrostatic interaction to obtain QDs hybridization probes for facilitating extremely sensitive detection of the human papillomavirus 16 nucleic acid (Fig. 4(a))[71]. The coupling of carbon QDs and TARMA-modified DNA resulted in fluorescence quenching, and complementary hybridization between the target nucleic acid and DNA probe induced the fluorescence recovery. Because of the strong quenching efficiency of TARMA by carbon QDs, this method was found to display high sensitivity. To further improve the sensitivity, Tianet al. enabled fluorescence resonance energy transfer (FRET) from DNA functionalized QDs to carbon nanotube (CNT) through π–π stacking interaction (Fig. 4(b))[18]. CNT was considered as an effective receptor in FRET system due to its strong absorption capacity, thereby resulting in high signal-to-noise ratio. After hybridizing with H5N1 nucleic acid, the generation of the rigid DNA double strand blocked the FRET effect and caused fluorescence recovery.
![(Color online) Construction of the nucleic acid hybridization probe for virus detection. (a) QD-DNA complexes were prepared by electrostatic interaction between Dabcyl modified DNA and carbon QDs[71]. (b) π–π stacking interactions between CNTs and aromatic nucleotide in DNA functionalized CdTe QDs[18]. (c) The conjugation of the plasmonic Au NPs and CdSeS QDs through the covalent coupling to form the plasmonic Au NP-QDs nanohybrids for Zika virus detection[75]. (d) The preparation of QD-NBs and the detection of HIV-1 genomic RNAs detection in the living cell[66]. Modified with permission from (a) Ref. [68] 2022 Elsevier B.V. All rights reserved, (b) Ref. [69] 2012 Elsevier B.V. All rights reserved, (c) Ref. [72] 2017 Elsevier B.V. All rights reserved and (d) Ref. [63] Copyright 2019 American Chemical Society.](/Images/icon/loading.gif)
Figure 4.(Color online) Construction of the nucleic acid hybridization probe for virus detection. (a) QD-DNA complexes were prepared by electrostatic interaction between Dabcyl modified DNA and carbon QDs[71]. (b) π–π stacking interactions between CNTs and aromatic nucleotide in DNA functionalized CdTe QDs[18]. (c) The conjugation of the plasmonic Au NPs and CdSeS QDs through the covalent coupling to form the plasmonic Au NP-QDs nanohybrids for Zika virus detection[75]. (d) The preparation of QD-NBs and the detection of HIV-1 genomic RNAs detection in the living cell[66]. Modified with permission from (a) Ref. [68] 2022 Elsevier B.V. All rights reserved, (b) Ref. [69] 2012 Elsevier B.V. All rights reserved, (c) Ref. [72] 2017 Elsevier B.V. All rights reserved and (d) Ref. [63] Copyright 2019 American Chemical Society.
The specificity and reproducibility of the nucleic acid detection need to be enhanced due to the relatively weak binding of electrostatic and π–π interaction. Nanobeacon is prepared by covalent coupling, which can enhance the binding of QD and quencher for the specific and reproducible detection of viral nucleic acid. The commonly used quenchers include Cy5[72], black hole quencher (BHQ)[73], and Au NPs[74]. Adegokeet al. assembled the BHQ-DNA modified QDs on the surface of Au-NPs, and the fluorescence of QDs was quenched by BHQ without targeting the nucleic acid (Fig. 4(c))[75]. After hybridization with Zika RNA, QD fluorescence was recovered and further enhanced under the effect of the surface plasmon resonance with Au NPs, with the detection limit being as low as 1.7 copies/mL. In addition, to avoid the influence of covalent coupling on the properties of QDs, our group has constructed BHQ-DNA functionalized QDs through the interaction of Cd2+ and phosphorothioate in BHQ-DNA (Fig. 4(d))[66]. This method did not affect the fluorescence of QDs, and the amount of DNA modified on QDs can be precisely controlled by the number of phosphorothioate groups. The developed QD nanobeacon was found to be suitable for imaging a single HIV-1 RNA in the latent infected cells, thus providing a platform for the analysis of single copy nucleic acid in the live cells.
3.1.2. Nucleic acid amplification
Nucleic acid amplification is a method that can amplify the nucleic acid to be evaluated through the catalyzation of the nuclease or nucleic acid self-assembly and then can aid in its detection. Nucleic acid amplification has been divided into variable temperature amplification and isothermal amplification according to the temperature control. Polymerase chain reaction (PCR) is considered as the most common variable temperature amplification method because of its high amplification efficiency[76]. For instance, Chenet al. used biotin-primer and digoxin-primer/TAMRA-primer to amplify SARS-CoV-2 N gene /influenza B virus by PCR, and the generated amplicon served as a potential bridge between the digoxin antibody/TAMRA antibody modified test strip and streptavidin-QDs (SA-QDs) to facilitate the fluorescence detection of SARS-CoV-2/influenza virus (Fig. 5(a))[77]. However, compared with PCR, isothermal amplification possesses the advantages of rapidity, high efficiency, good specificity and lack of requirement of variable temperature instrument. Loop-mediated isothermal amplification (LAMP) has received considerable attention in nucleic acid detection due to its simple temperature control technique and short detection time[78]. It is characterized by designing four distinct primers for six regions on the target gene, by using DNA polymerase with chain replacement activity to amplify the nucleic acid under constant temperature condition, which can achieve about 109 times amplification in 15–60 min[79]. Leeet al. amplified the nucleic acid of SARS-CoV-2 by LAMP, which resulted in the negatively charged polyphosphate (Mg2P2O7), and then aggregated with positively charged cysteamine modified CdSeS/ZnS QDs lead to fluorescence quenching of QDs (Fig. 5(b))[80]. The fluorescence decrease observed in QDs could be used for SARS-CoV-2 detection.
![(Color online) Distinct kinds of nucleic acid amplification method for virus detection. (a) The testing workflow of a rapid PCR lateral flow assay for the simultaneous detection of SARS- CoV-2 and influenza B virus. The nucleic acid was amplified by the rapid water bath RT-PCR, and the labeled amplicons were detected by using test strips[77]. (b) The one-step loop-mediated isothermal amplification (LAMP) assay with cysteamine-modified CdSeS/ZnS QDs for SARS-CoV-2 detection. LAMP produced the negatively charged polyphosphate, which can aggregate with the positively charged cysteamine modified CdSeS/ZnS QDs lead to fluorescence quenching of QDs[80]. (c) HCV RNA ultrasensitive determination based on CHA-induced the in-situ degradation of silver nanocomposite in GQD/Ag NCs to recover the fluorescence[82]. Modified with permission from (a) Ref. [74] Copyright The Royal Society of Chemistry 2022, (b) Ref. [77] Copyright 2022 American Chemical Society and (c) Ref. [79] 2022 Elsevier B.V. All rights reserved.](/Images/icon/loading.gif)
Figure 5.(Color online) Distinct kinds of nucleic acid amplification method for virus detection. (a) The testing workflow of a rapid PCR lateral flow assay for the simultaneous detection of SARS- CoV-2 and influenza B virus. The nucleic acid was amplified by the rapid water bath RT-PCR, and the labeled amplicons were detected by using test strips[77]. (b) The one-step loop-mediated isothermal amplification (LAMP) assay with cysteamine-modified CdSeS/ZnS QDs for SARS-CoV-2 detection. LAMP produced the negatively charged polyphosphate, which can aggregate with the positively charged cysteamine modified CdSeS/ZnS QDs lead to fluorescence quenching of QDs[80]. (c) HCV RNA ultrasensitive determination based on CHA-induced the in-situ degradation of silver nanocomposite in GQD/Ag NCs to recover the fluorescence[82]. Modified with permission from (a) Ref. [74] Copyright The Royal Society of Chemistry 2022, (b) Ref. [77] Copyright 2022 American Chemical Society and (c) Ref. [79] 2022 Elsevier B.V. All rights reserved.
In addition, enzyme-free amplification techniques have been also widely used in nucleic acid detection. Catalyzed hairpin assembly (CHA) is achieved by the target-induced DNA hairpin self-assembly and has been proven to be an effective enzyme-free amplification technique[81]. Liet al. described a novel colorimetric determination of hepatitis C virus (HCV) RNA based on the magnetic separation and catalytic hairpin assembly (CHA) (Fig. 5(c))[82]. Hairpin 1 (H1) was modified onto MBs and opened by the target HCV RNA, which further unfolded the biotin-hairpin 2 (H2) to release the target HCV RNA for further hybridization. Glucose oxidase modified with SA was bound onto biotin-H2, which can catalyze the glucose to produce H2O2 to induce in situ degradation of the silver nanocomposite in graphene QDs silver nanocomposites (GQD/Ag NCs). GQDs fluorescence recovered after interaction of H2O2 and silver nanocomposite was used for HCV RNA detection. This method is simple, stable, and sensitive with detection limit of 24.8 pM, which can be effectively used for the rapid screening of virus infection.
3.1.3. Signal enhancement of probe
In addition to amplifying the various targets present in sample, the reporter signal enhancing is another effective method for augmenting sensitivity. Assembly of QDs can effectively improve the reporter signals, such as liposome coating QDs and QDs layer-by-layer assembly. Zhouet al. described a novel approach for the sensitive detection of low-abundance HIV DNA by using liposome coating QDs (Fig. 6(a))[83]. Liposomes were used to encapsulate the QDs and then modified with DNA to form the DNA-L/QD complex. Thereafter, the target HIV DNA can link the DNA-L/QD complex and DNA modified magnetic beads (MBs). which can be detected with single-particle detection techniques. This method allows for the optimal detection of HIV DNA at attomolar concentration without any target amplification, which can reach up to 3 orders of magnitude in comparison with that of single QDs-based method. Cui et al. developed a signal enhancement method for the detection of HIV DNA via a QD layer-by-layer assembled polystyrene microspheres (PS) to simplify the assembly process (Fig. 6(b))[84]. PS was used as the core and was able to attract the biotin-QDs by electrostatic interaction, and then could react with SA-QDs to produce layer-by-layer QDs assembly on the surface of the PS (PS-QDs). In the presence of the target HIV DNA, biotin-DNA conjugated with the PS-QDs as the detection reporter was bound onto 96-well plate immobilized with captured probes to produce the fluorescence signals, which could reach ~10 amol of target detection. This method has been found to be simple and controllable.
![(Color online) Signal enhancement methods of the probes for virus detection. (a) Detection of attomolar HIV-1 DNA using encapsulated QDs-encapsulated liposome complexes and single-particle detection techniques[83]. (b) The simultaneous detection of HIV-1 and HIV-2 via QD layer-by-layer assembled polystyrene microsphere by the interaction of SA and biotin[84]. (c) One-step simultaneous detection of EV71 infection-related miRNA based on DSN-assisted target amplification followed by the magnetic separation[88]. (d) The detection of African swine fever virus by combining CRISPR-Cas mediated nucleic acid probe cleavage and highly sensitive reporter of QDs[90]. (e) SARS-CoV-2 detection by combination of reverse-transcription recombinase-aided amplification assisted-CRISPR/Cas13a with QDs microspheres[91]. Modified with permission from (a) Ref. [80] Copyright 2013 American Chemical Society, (b) Ref. [81] Copyright 2015 John Wiley & Sons, Ltd, (c) Ref. [82] Copyright 2020 American Chemical Society, (d) Ref. [84] Copyright 2020 American Chemical Society and (e) Ref. [85] 2022 Elsevier B.V. All rights reserved.](/Images/icon/loading.gif)
Figure 6.(Color online) Signal enhancement methods of the probes for virus detection. (a) Detection of attomolar HIV-1 DNA using encapsulated QDs-encapsulated liposome complexes and single-particle detection techniques[83]. (b) The simultaneous detection of HIV-1 and HIV-2 via QD layer-by-layer assembled polystyrene microsphere by the interaction of SA and biotin[84]. (c) One-step simultaneous detection of EV71 infection-related miRNA based on DSN-assisted target amplification followed by the magnetic separation[88]. (d) The detection of African swine fever virus by combining CRISPR-Cas mediated nucleic acid probe cleavage and highly sensitive reporter of QDs[90]. (e) SARS-CoV-2 detection by combination of reverse-transcription recombinase-aided amplification assisted-CRISPR/Cas13a with QDs microspheres[91]. Modified with permission from (a) Ref. [80] Copyright 2013 American Chemical Society, (b) Ref. [81] Copyright 2015 John Wiley & Sons, Ltd, (c) Ref. [82] Copyright 2020 American Chemical Society, (d) Ref. [84] Copyright 2020 American Chemical Society and (e) Ref. [85] 2022 Elsevier B.V. All rights reserved.
Amplification based on the nuclease, such as duplex specific nuclease (DSN) and clustered regularly interspaced short palindromic repeat (CRISPR)-associated (Cas) nuclease, could enhance the detection sensitivity and simplify the process[85-87]. For instance, Wang et al. developed one-step method for enterovirus 71 (EV71) infection-related miRNA based on DSN-assisted target amplification and magnetic separation (Fig. 6(c))[88]. DNA functionalized QDs were covalently coupled onto the magnetic beads, which could be effectively released by the target hybridization and DSN-assisted DNA cleavage. After the magnetic separation, the released QDs were used for EV71 infection-related miRNA concentration determination. A novel signal amplification method was designed for virus detection based on CRISPR system. However, due to the recognition and cleavage of the target nucleic acid, CRISPR and CRISPR-Cas systems, discovered as adaptive immune systems for bacteria against invading nucleic acids, have gained significant attention in biosensor[89]. For example, Baoet al. developed a novel method for the detection of African swine fever virus by combining CRISPR-Cas mediated nucleic acid probe cleavage and highly sensitive reporter of QDs (Fig. 6(d))[90]. In the present of target, biotin ssDNA probe could be cleaved by activated Cas12a, but SA-QDs were unable to conjugate with MB, which can be detected by portable instrument after the magnetic separation. This method has displayed high sensitivity and could be used for the visual detection coupled with easy operation. Combining CRISPR-based signal amplification and QD assembly-based signal enhancement could further improve the sensitivity. Zhanget al. proposed another method for SARS-CoV-2 detection which used the combination of isothermal amplification-CRISPR/Cas13a with QDs microspheres (Fig. 6(e))[91]. SARS-CoV-2 Spike gene was amplified by reverse-transcription recombinase-aided amplification and transcribed into RNA to specifically activate the cleavage of Cas13a. After Cas13a activated cleavage of biotin-RNA-FAM, QDs microspheres were not able to bind and hence not detected on the test strip. The SARS-CoV-2 Spike gene could be detected according to the QDs signals on strip, which is fast, sensitive, and specific with no need for complicated instruments. These advantages demonstrate its potential application for in situ screening of SARS-CoV-2 during pandemic, especially in the remote and underdeveloped areas with limited equipment and resources.
3.2. Immunoassays for virus infection diagnosis
3.2.1. Heterogeneous immunoassay
In heterogeneous detection system, the antigen-antibody complex is physically separated from the free components. According to the separation interface, it has been primarily divided into enzyme-linked immunosorbent assay (ELISA), magnetic bead-based immunoassay (MBIA), and lateral-flow immunochromatographic assay (LFIA). Among them, ELISA can combine the specific antigen-antibody recognition and facilitate efficient biocatalysis of enzymes[92]. The output signals are generated by catalyzing the substrate through the enzyme coupled with an antibody or antigen. Because of its high throughput, low cost, and straightforward readout, it is the most widely used immunoassay. The sensitivity of ELISA system based on the colorimetric signal is too low to detect low-level proteins, while fluorescence signal can significantly increase the sensitivity by 1–2 orders of magnitude[93], and QD is considered as a suitable candidate for fluorescence reporter. Wuet al. proposed an ELISA based method for the detection of hepatitis B virus based on glucose oxidase (GOx)-induced fluorescence quenching of CdTe QDs (Fig. 7(a))[94]. In this system, the GOx was coupled onto polystyrene microplates through immunoreaction of hepatitis B virus with antibody to catalyze the glucose to generate H2O2. The hepatitis B virus was detected by the fluorescence quenching of H2O2-sensitvie CdTe QDs. This method displayed high detection sensitivity, which was approximately 430-folds lower than that of HRP-catalyzed conventional ELISA. Zhouet al. reported a dual-mode rabies virus detection using dendritic silica nanospheres (DSNs) with the densely packed QDs and HRP-labeled antibody to substantially broaden the application scenarios of ELISA. DSNs were prepared for QDs encapsulation and then covalently coupled with HRP-labeled antibody. As shown inFig. 7(b), the sandwich-structure immunocomplexes were mainly formed between rabies virus antigen and antibody. After addition of H2O2 and TMB, an obvious color change was observed due to the HRP-catalyzed TMB oxidation[95]. Furthermore, rabies virus can also be detected by measuring the fluorescence intensity of QDs embedded DSNs. This method displayed wider linear range and nearly 2 orders of magnitude lower than that of the conventional ELISA. This method using DSNs for the colorimetry-based prescreening and fluorescence-based quantitative detection might pave the way for virus-related disease diagnosis. Enzyme labeling is usually used for signal amplification in ELISA system, but its stability is relatively low and its activity is vulnerable to environmental interference. To solve these limitation, Zhaoet al. developed a DNA label method for immunoassay of HIV-1 p24 antigen (Fig. 7(c))[96]. In this method, the DNA was conjugated onto indium tin oxide (ITO) electrode after the sandwich immunobinding, which can be released after treating with NaOH. The subsequent depurination of the oligonucleotide strands by H2SO4 can enable the oxidation of free nucleobases (poly G) at a CdTe QDs modified ITO transducer to produce the photoelectrochemical signal. The DNA labeling displays better stability, although the sensitivity needs to be further improved.
![(Color online) Heterogeneous immunoassay. ELISA: (a) Fluorescence ELISA based on GOx-induced fluorescence quenching of H2O2-sensitvie CdTe QDs for the surface antigen detection of hepatitis B[94]; (b) Dual-modal immunoassay of rabies virus based on QDs and HRP-Ab co-modified silica nanospheres. The colorimetric signal was obtained by HRP-catalyzed TMA oxidation and the fluorescence signal was captured from loading QDs[95]; (c) Photoelectrochemical immunoassay of HIV-1 p24 antigen using DNA as ELISA label tag. Following the sandwich immunobinding, the DNA tags could be released and subsequent dipurinization of oligonucleotide strands could enable the oxidation of free nucleobases at a CdTe QDs modified ITO transducer[96]. MBIA: (d) Magnetic immunoassay of H1N1 virus based on immune-complex formation between QD-embedded silica nanoparticle and magnetic beads[97]; (e) The preparation of CdSeTeS QD/AuNPs nanocomposites and the close covalent attachment of AuNPs with CdSeTeS QDs effectively quenched the fluorescence signal which was recovered after NoV-LPs entrapment[98]; (f) Digital single virus immunoassay for the multiplex virus detection using fluorescent magnetic multifunctional nanospheres (FMNs) as both capture carriers and signal labels[100]. LFIA: (g) The fabrication of SiTQD probes and their application in ICA-based biosensor for the simultaneous detection of SARS-CoV-2 and FluA[102]; (h) SARS-CoV-2 spike protein detection by fabricating dual-functional SiO2@Au/QD labels. The SiO2@Au/QD labels were constructed by the layer-by-layer electrostatic interaction of SiO2@PEI, Au NPs, and QDs[103]. Modified with permission from (a) Ref. [88] 2018 Elsevier B.V. All rights reserved, (b) Ref. [89] Copyright 2020 American Chemical Society, (c) Ref. [90] Copyright 2015 American Chemical Society, (d) Ref. [91] 2020 Elsevier B.V. All rights reserved, (e) Ref. [92] 2018 Elsevier B.V. All rights reserved, (f) Ref. [94] Copyright 2019 American Chemical Society, (g) Ref. [96] 2021 Elsevier B.V. All rights reserved and (h) Ref. [97] 2021 Elsevier B.V. All rights reserved.](/Images/icon/loading.gif)
Figure 7.(Color online) Heterogeneous immunoassay. ELISA: (a) Fluorescence ELISA based on GOx-induced fluorescence quenching of H2O2-sensitvie CdTe QDs for the surface antigen detection of hepatitis B[94]; (b) Dual-modal immunoassay of rabies virus based on QDs and HRP-Ab co-modified silica nanospheres. The colorimetric signal was obtained by HRP-catalyzed TMA oxidation and the fluorescence signal was captured from loading QDs[95]; (c) Photoelectrochemical immunoassay of HIV-1 p24 antigen using DNA as ELISA label tag. Following the sandwich immunobinding, the DNA tags could be released and subsequent dipurinization of oligonucleotide strands could enable the oxidation of free nucleobases at a CdTe QDs modified ITO transducer[96]. MBIA: (d) Magnetic immunoassay of H1N1 virus based on immune-complex formation between QD-embedded silica nanoparticle and magnetic beads[97]; (e) The preparation of CdSeTeS QD/AuNPs nanocomposites and the close covalent attachment of AuNPs with CdSeTeS QDs effectively quenched the fluorescence signal which was recovered after NoV-LPs entrapment[98]; (f) Digital single virus immunoassay for the multiplex virus detection using fluorescent magnetic multifunctional nanospheres (FMNs) as both capture carriers and signal labels[100]. LFIA: (g) The fabrication of SiTQD probes and their application in ICA-based biosensor for the simultaneous detection of SARS-CoV-2 and FluA[102]; (h) SARS-CoV-2 spike protein detection by fabricating dual-functional SiO2@Au/QD labels. The SiO2@Au/QD labels were constructed by the layer-by-layer electrostatic interaction of SiO2@PEI, Au NPs, and QDs[103]. Modified with permission from (a) Ref. [88] 2018 Elsevier B.V. All rights reserved, (b) Ref. [89] Copyright 2020 American Chemical Society, (c) Ref. [90] Copyright 2015 American Chemical Society, (d) Ref. [91] 2020 Elsevier B.V. All rights reserved, (e) Ref. [92] 2018 Elsevier B.V. All rights reserved, (f) Ref. [94] Copyright 2019 American Chemical Society, (g) Ref. [96] 2021 Elsevier B.V. All rights reserved and (h) Ref. [97] 2021 Elsevier B.V. All rights reserved.
Magnetic bead-based immunoassay (MBIA) is used to modify the MB and reporter with antibodies respectively, promote their binding with the target to form MB-antibody-target-antibody-reporter complex and remove the various impurities through the magnetic separation for signal detection. Joet al. constructed QDs-embedded silica nanoparticles, which showed 500-times stronger fluorescence in comparison to individual QDs (Fig. 7(d))[97]. In the present of target avian virus, antibody modified QDs-embedded silica nanoparticles were bound with antigen and antibody modified MBs, and the fluorescence intensity of QDs-embedded silica nanoparticles can be used for avian virus detection after the magnetic separation. The sensitivity of this MBIA method is about 2000 times better than the hemagglutination method (a typical method for influenza virus detection). In addition, Park’s group developed an innovative localized surface plasmon resonance (LSPR)-amplified magnetofluoroiimmunoassay for norovirus and influenza virus detection to further enhance the sensitivity (Fig. 7(e))[98]. Au NPs and MBs hybrid nanocomposite (AuNP/MNP) was synthesized and used for the magnetic separation and high localized surface plasmon resonance (LSPR) effect. In the present of target norovirus, antibody modified QDs could bind with the target norovirus as well as antibody modified AuNP/MNP, and the fluorescence of QDs was effectively enhanced through the LSPR energy transfer between AuNP/MNP and QDs after the magnetic separation. However, due to magnetic separation and high LSPR effect, this method could display high signal-to-noise, which is102-fold greater that of above ELISA. Digital immunoassays can divide analytes into microwells or emulsion droplets for independent signal amplification, thus resulting in sensitivity increase over traditional immunoassays[99]. Wu et al proposed a digital single virus immunoassay for the multiplex virus detection using fluorescent magnetic multifunctional nanospheres (FMNs) as both the capture carriers and signal labels, which prepared by the assembling MB and QDs through electrostatic interaction (Fig. 7(f))[100]. After sandwich immunoreaction, fluorescence images of 500 micropores were obtained. Furthermore, corresponding to with or without target virus, the micropores with or without fluorescence were denoted as “1” or “0” based on the digital analysis, respectively. The virus concentration can be calculated by the “1” probability. Green, yellow, and red fluorescent magnetic multifunctional nanospheres were used for H9N2, H1N1, and H7N9 avian influenza virus detection, respectively. This method displayed high sensitivity (0.02 pg/mL of detection limit), good specificity, and versality for multiplex detection.
LFIA is rapid easy to operate, low-cost, and point-of-care testing tool for virus infection diagnosis[101]. In conventional LFIA, capture and control antibodies are immobilized on the test as well as the control lines in the strip, and in the present of target antigen, antigen-antibody-conjugated Au NPs complexes are formed and could pass through the test line to generate the test signals by interacting with the capture antibody. Free antibody-conjugated Au NPs could then bind with the control antibody to verify the availability of the test strip. Due to high stability, easy preparation, and strong colorimetric signal, Au NPs have been commonly used as LFIA reporter. QDs have replaced the Au NPs as the new reporter and this modification is being considered as promising strategy to enhance the detection sensitivity of LFIA. Wang’s group developed a high-performance QDs nanobead fabricated by absorption of multilayers of QDs onto the SiO2 surface through electrostatic interaction of PEI-SiO2/PEI and QDs to produce Si@TQD. The detection antibody was modified onto the Si@TQD, which was used for the detection of SARS-CoV-2 antigen and influenza A virus (Fig. 7(g))[102]. This method displayed 2 orders of magnitude improvement in sensitivity compared with conventional Au NP-based LFIA. In another study, to enhance the repeatability and accuracy of LFIA, Hanet al. fabricated a dual-functional SiO2@Au/QD fluorescence labels by coating Au NPs and QDs onto SiO2. The detection antibody was modified onto SiO2@Au/QD, which was used as both colorimetric and fluorescence signals for SARS-CoV-2 spike protein detection (Fig. 7(h))[103]. The colorimetric signal was employed for the visual detection and rapid screening of SARS-CoV-2 infection, whereas the fluorescence signal was used for sensitive and quantitative detection of virus infection at the early stage, which displayed good repeatability, accuracy, and broad application range. Portable instruments to read the QDs fluorescence signal are highly in demand for providing diagnosis at home, while there are still some challenges to be solved, including portable excitation light source, and simple and accurate fluorescent reading system.
3.2.2. Homogeneous immunoassay
Because no separation and purification procedures are required, homogeneous immunoassays can enable a one-step, rapid, and direct detection of different analytes. Homogenous immunoassays are mainly based on the fluorescence resonance energy transfer (FRET) from antigen/antibody modified QDs to quencher, which could be blocked due to antibody-antigen interactions-induced distance enlargement between the donor (QDs) and acceptor (quencher). A variety of molecules have been used as acceptors, such as metal-ligand complex, GO, and Au NPs. Chenet al. developed a homogeneous immunoassay for enterovirus 71 using Ru(bpy)2(mcbpy-O-Su-ester)(PF6)2-antibody (Ru-Ab) as the quencher of QDs fluorescence and the capture probe of the target enterovirus 71 (EV71). As shown inFig. 8(a), Ru-Ab can bind with QDs through the electrostatic interaction and quench the QDs fluorescence[104]. The QDs were then set free and the fluorescence was recovered after the binding of Ru-Ab with target EV71. This method could not only provide rapid and precise quantitative determination of EV71 by fluorescence intensity but also could be applied in semi- \quantitative EV71 detection by digital visualization, which could yield a simple, fast, highly selective approach for virus infection diagnosis. In another report, combining the wide absorption range of GO and multiplex emission of QDs, Chenet al. proposed a method for EV71 and coxsackievirus B3 (CVB3) simultaneous detection using antibody modified QDs and GO (Fig. 8(b))[105]. Green and red QDs were modified with EV71 antibody and CVB3 antibody, both of which were quenched by GO through π–π stacking interaction of antibody and GO. After adding the target virus, the interaction of antibody modified QDs with GO was broken up, and the fluorescence of QDs was recovered. Due to the broad excitation and narrow emission of QDs, this sensor was used for the multiplex detection using a single-wavelength excitation light and can provide a novel option for screening of virus species or subtype differentiation both simply and effectively. Nasrinet al. developed a method for norovirus detection by combining LSPR behavior of Au NPs and FRET from QDs to Au NPs to markedly improve the detection sensitivity (Fig. 8(c))[98]. Without target virus, QDs fluorescence was quenched by Au NPs via FRET due to the close interaction of QDs and Au NPs. However, in the present of target virus, steric hindrance blocked the FRET from QDs to Au NPs, and further triggered the fluorescence enhancement of QDs via target-induced the LSPR from Au NPs to QDs. This method provided synergistic fluorescence enhancement of QDs by combining FRET and LSPR, which displayed pretty high sensitivity which was 100-fold greater than that of commercial ELISA kit.
![(Color online) Homogeneous immunoassay. FRET: (a) EV71 detection caused by the interaction of QDs and Ru-Ab complex[104]; (b) EV71 and CVB3 simultaneous determination by the interaction of dual-color QDs-Ab complex and GO[105]; (c) Norovirus biosensor developed by combining LSPR from Au NPs to CdSeTeS QDs which blocked FRET from QDs to Au NPs[98]. QDSMs: (d) QDs-loaded spore-based monodisperse microparticles for immunoassay of parvovirus antibody via flow cytometry[106]. UCAD: (e) The workflow for the proximity-induced CRISPR-based SRAS-CoV-2 antibody detection. Proximity binding of the DNA probes to the SRAS-CoV-2 antibody can induce the dsDNA formation, primer extension and RPA, and CRISPR/Cas12a system to produce the fluorescence signal[108]. Modified with permission from (a) Ref. [98] Copyright 2011 American Chemical Society, (b) Ref. [99] Copyright 2012 American Chemical Society, (c) Ref. [92] 2015 Elsevier B.V. All rights reserved, (d) Ref. [100] Copyright 2022 The Author(s) Springer Nature and (e) Ref. [102] Copyright 2022 The Author(s) Springer Nature.](/Images/icon/loading.gif)
Figure 8.(Color online) Homogeneous immunoassay. FRET: (a) EV71 detection caused by the interaction of QDs and Ru-Ab complex[104]; (b) EV71 and CVB3 simultaneous determination by the interaction of dual-color QDs-Ab complex and GO[105]; (c) Norovirus biosensor developed by combining LSPR from Au NPs to CdSeTeS QDs which blocked FRET from QDs to Au NPs[98]. QDSMs: (d) QDs-loaded spore-based monodisperse microparticles for immunoassay of parvovirus antibody via flow cytometry[106]. UCAD: (e) The workflow for the proximity-induced CRISPR-based SRAS-CoV-2 antibody detection. Proximity binding of the DNA probes to the SRAS-CoV-2 antibody can induce the dsDNA formation, primer extension and RPA, and CRISPR/Cas12a system to produce the fluorescence signal[108]. Modified with permission from (a) Ref. [98] Copyright 2011 American Chemical Society, (b) Ref. [99] Copyright 2012 American Chemical Society, (c) Ref. [92] 2015 Elsevier B.V. All rights reserved, (d) Ref. [100] Copyright 2022 The Author(s) Springer Nature and (e) Ref. [102] Copyright 2022 The Author(s) Springer Nature.
Zhanget al. reported a novel method for the detection of parvovirus antibody by QDs-loaded spore-based monodisperse microparticles (QDSMs) to improve both output and sensitivity (Fig. 8(d))[106]. Antigen modified green QDSMs were constructed by embedding QDs onto the surface of spore-based monodisperse microparticles and modifying with parvovirus. In the presence of the target antibody, antigen modified green QDSMs were bound with the target antibody and Alexa Fluor 647-IgG to form QDSM-detection antibody-Alexa Fluor 647-IgG, which were used as model system to detect the parvovirus antibody via using flow cytometry. A variety of barcodes can be obtained by potential interaction of QDSMs with the distinct colors upon using emitting fluorescence dye, which displayed enormous potential in high-throughput immunoassays. Furthermore, due to the advantages such as simplicity, low cost, high throughout and eco-friendliness, this method may find wide applications in rapid disease screening. Recently, many homogeneous immunoassays based on the nucleic acid amplification and protein complementation assays have been developed, such as proximity ligation and split tripart Nanoluciferase[107]. Liet al. reported an ultrasensitive CRISPR-based antibody detection (UCAD) assay that can effectively translate the detection of anti-SARS-CoV-2 antibodies into CRISPR-based nucleic acid detection by combining proximity ligation, T4 polymerase-based amplification, and RPA-assisted CRISPR/Cas12a system. As shown inFig. 8(e), DNA modified detection antibody and DNA modified region binding domain were found to bind with anti-SARS-CoV-2 antibodies to induce the proximity binding of DNA probes and the formation of dsDNA assisted by T4 polymerase[108]. Recombinase polymerase amplification of dsDNA can cause amplicon to trigger the subsequent indiscriminate single-stranded DNase activity of Cas12a to produce the fluorescence signal. This method could translate antibody into the nucleic acid amplification, which displayed 10,000 times more sensitive than the commercial ELISA and could be used for rapidly tracking the antibody levels changes with ultrahigh sensitivity in infected or vaccinated individuals. Integrated with QDs, these methods could provide higher sensitivity and better portability due to the high quantum yield of QDs.
4. Conclusion
This review article mainly discussed the strategies employed for the synthesis and biofunctionalization of QDs for virus detection. The Covid-19 pandemic caused by the virus has attracted widespread attention around the world. The traditional virus detection method is performed in the laboratory by using a large clinical analyzer, such as PCR and ELISA. These methods require the availability of trained technicians and long detection time. The rapid development of QDs has significantly improved the design and manufacture of novel devices for biosensing. A variety of methods based on QDs for virus detection to substantially simplify operation and enhance detection sensitivity, including nucleic acid hybridization, nucleic acid amplification, probe signal enhancement, and enzyme linked amplification have been developed. However, several challenges must be addressed to facilitate their better performance in controlling the outbreak of pandemic diseases. 1) The potential toxicity of the semiconductor QDs can effectively limit their clinic applications in diseases diagnosis. Hence, the preparation of relatively less and even non-toxic QDs are required for the construction of safe and eco-friendly biosensors. In addition, some lab-made immature QDs are hardly produced at large-scale, so it is necessary to optimize simple and middle method for preparation of large-scale QDs. 2) For the viral nucleic acid detection, conventional PCR or LAMP are time-consuming, whereas application of the new emerging technology, such as CRISPR, is associated with high cost. In addition, viral nucleic acid extraction is unavoidable and complex. The combination of the nucleic acid free-extraction method and QD-based virus biosensor could significantly improve the operability of molecular diagnosis. 3) For immunoassays, ELISA is time-consuming, LFIA has low sensitivity, however there only are few effective methods available that can integrate both high speed and sensitivity. Some synthetic biology sensors have been developed that can exhibit rapidity, specificity, as well as robustness and thus integrating them with QDs as reporter could further enhance the detection sensitivity.
References
[1] Y A Kim, T M Przytycka. The language of a virus. Science, 371, 233(2021).
[2] S Mukherjee et al. Before virus, after virus: A reckoning. Cell, 183, 308(2020).
[3] J Castilla, P Saá, C Soto. Detection of prions in blood. Nat Med, 11, 982(2005).
[4] P Kukura, H Ewers, C Müller et al. High-speed nanoscopic tracking of the position and orientation of a single virus. Nat Methods, 6, 923(2009).
[5] M Xiao, F Tian, X Liu et al. Virus detection: From state-of-the-art laboratories to smartphone-based point-of-care testing. Adv Sci, 9, e2105904(2022).
[6] S Hassanpour et al. Recent trends in rapid detection of influenza infections by bio and nanobiosensor. Trac Trends Anal Chem, 98, 201(2018).
[7] W J Wiersinga, H C Prescott. What is COVID-19?. JAMA, 324, 816(2020).
[8] N J Matheson, P J Lehner. How does SARS-CoV-2 cause COVID-19?.. Science, 369, 510(2020).
[9] J Q Deng, S Zhao, Y Liu et al. Nanosensors for diagnosis of infectious diseases. ACS Appl Bio Mater, 4, 3863(2021).
[10] J Abbasi. Combining rapid PCR and antibody tests improved COVID-19 diagnosis. JAMA, 324, 1386(2020).
[11] K Deshpande, U Pt, O Kaduskar et al. Performance assessment of seven SARS-CoV-2 IgG enzyme-linked immunosorbent assays. J Med Virol, 93, 6696(2021).
[12] R Krajewski et al. Update on serologic testing in COVID-19. Clin Chimica Acta, 510, 746(2020).
[13] M L Song et al. Pathogenic virus detection by optical nanobiosensors. Cell Rep Phys Sci, 2, 100288(2021).
[14] M Abdolhosseini et al. A review on colorimetric assays for DNA virus detection. J Virol Methods, 301, 114461(2022).
[15] M Nasrollahzadeh, M Sajjadi, G J Soufi et al. Nanomaterials and nanotechnology-associated innovations against viral infections with a focus on coronaviruses. Nanomaterials, 10, 1072(2020).
[16] B B Lou, Y F Liu, M L Shi et al. Aptamer-based biosensors for virus protein detection. Trac Trends Anal Chem, 157, 116738(2022).
[17] Ž Jelen, P Majerič, M Zadravec et al. Study of gold nanoparticles’ preparation through ultrasonic spray pyrolysis and lyophilisation for possible use as markers in LFIA tests. Nanotechnol Rev, 10, 1978(2021).
[18] J P Tian, H M Zhao, M Liu et al. Detection of influenza A virus based on fluorescence resonance energy transfer from quantum dots to carbon nanotubes. Anal Chimica Acta, 723, 83(2012).
[19] C W Wang, C G Wang, X L Wang et al. Magnetic SERS strip for sensitive and simultaneous detection of respiratory viruses. ACS Appl Mater Interfaces, 11, 19495(2019).
[20] J Lee, S R Ahmed, S Oh et al. A plasmon-assisted fluoro-immunoassay using gold nanoparticle-decorated carbon nanotubes for monitoring the influenza virus. Biosens Bioelectron, 64, 311(2015).
[21] W D Zhou, J J Coleman. Semiconductor quantum dots. Curr Opin Solid State Mater Sci, 20, 352(2016).
[22] de Arquer F P García, D V Talapin, V I Klimov et al. Semiconductor quantum dots: Technological progress and future challenges. Science, 373, eaaz8541(2021).
[23] L J Zhang, L Xia, H Y Xie et al. Quantum dot based biotracking and biodetection. Anal Chem, 91, 532(2019).
[24] G V Lisichkin, A Y Olenin. Synthesis of surface-modified quantum dots. Russ Chem Bull, 69, 1819(2020).
[25] X H Chang, J Zhang, L H Wu et al. Research progress of near-infrared fluorescence immunoassay. Micromachines, 10, 422(2019).
[26] M Pastucha, Z Farka, K Lacina et al. Magnetic nanoparticles for smart electrochemical immunoassays: A review on recent developments. Mikrochim Acta, 186, 312(2019).
[27] M Stanisavljevic, S Krizkova, M Vaculovicova et al. Quantum dots-fluorescence resonance energy transfer-based nanosensors and their application. Biosens Bioelectron, 74, 562(2015).
[28] Q Zhao, D Lu, G Y Zhang et al. Recent improvements in enzyme-linked immunosorbent assays based on nanomaterials. Talanta, 223, 121722(2021).
[29] A M Wagner, J M Knipe, G Orive et al. Quantum dots in biomedical applications. Acta Biomater, 94, 44(2019).
[30] J Zhou, Y Yang, C Y Zhang. Toward biocompatible semiconductor quantum dots: From biosynthesis and bioconjugation to biomedical application. Chem Rev, 115, 11669(2015).
[31] R Gill, M Zayats, I Willner. Semiconductor quantum dots for bioanalysis. Angew Chem Int Ed, 47, 7602(2008).
[32] P Reiss, M Carrière, C Lincheneau et al. Synthesis of semiconductor nanocrystals, focusing on nontoxic and earth-abundant materials. Chem Rev, 116, 10731(2016).
[33] D Zhao, Z K He, W H Chan et al. Synthesis and characterization of high-quality water-soluble near-infrared-emitting CdTe/CdS quantum dots capped byN-acetyl-l-cysteine via hydrothermal method. J Phys Chem C, 113, 1293(2009).
[34] J B Blanco-Canosa, M Wu, K Susumu et al. Recent progress in the bioconjugation of quantum dots. Coord Chem Rev, 263/264, 101(2014).
[35] A M Salaheldin, J Walter, P Herre et al. Automated synthesis of quantum dot nanocrystals by hot injection: Mixing induced self-focusing. Chem Eng J, 320, 232(2017).
[36] J Y Park, D W Jeong, K M Lim et al. Multimodal luminescence properties of surface-treated ZnSe quantum dots by Eu. Appl Surf Sci, 415, 8(2017).
[37] W T Wang, A Kapur, X Ji et al. Photoligation of an amphiphilic polymer with mixed coordination provides compact and reactive quantum dots. J Am Chem Soc, 137, 5438(2015).
[38] Z X Jiang, K Matras-Postolek, P Yang. Hydrophobic CdSe and CdTe quantum dots: Shell coating, shape control, and self-assembly. RSC Adv, 6, 25656(2016).
[39] O Adegoke, M W Seo, T Kato et al. An ultrasensitive SiO2-encapsulated alloyed CdZnSeS quantum dot-molecular beacon nanobiosensor for norovirus. Biosens Bioelectron, 86, 135(2016).
[40] N Q Zhan, G Palui, J P Merkl et al. Bio-orthogonal coupling as a means of quantifying the ligand density on hydrophilic quantum dots. J Am Chem Soc, 138, 3190(2016).
[41] P Yang, M Ando, N Murase. Controlled self-assembly of hydrophobic quantum dots through silanization. J Colloid Interface Sci, 361, 9(2011).
[42] Y He, H T Lu, L M Sai et al. Microwave synthesis of water-dispersed CdTe/CdS/ZnS core-shell-shell quantum dots with excellent photostability and biocompatibility. Adv Mater, 20, 3416(2008).
[43] N Gaponik, D V Talapin, A L Rogach et al. Thiol-capping of CdTe nanocrystals: An alternative to organometallic synthetic routes. J Phys Chem B, 106, 7177(2002).
[44] M Y Mou, Y Wu, Q Q Niu et al. Aggregation-induced emission properties of hydrothermally synthesized Cu-In-S quantum dots. Chem Commun, 53, 3357(2017).
[45] D Zhao, Y Fang, H Y Wang et al. Synthesis and characterization of high-quality water-soluble CdTe: Zn2+ quantum dots capped byN-acetyl-l-cysteineviahydrothermal method. J Mater Chem, 21, 13365(2011).
[46] C L Zhang, J Yan, C Liu et al. One-pot synthesis of DNA-CdTe: Zn2+ nanocrystals using Na2TeO3 as the Te source. ACS Appl Mater Interfaces, 6, 3189(2014).
[47] K Nekolla, K Kick, S Sellner et al. Influence of surface modifications on the spatiotemporal microdistribution of quantum dotsIn vivo. Small, 12, 2641(2016).
[48] G B Mao, W Q Peng, S B Tian et al. Dual-protein visual detection using ratiometric fluorescent probe based on Rox-DNA functionalized CdZnTeS QDs. Sens Actuat B, 283, 755(2019).
[49] Y X Ma, G B Mao, G Q Wu et al. A novel nano-beacon based on DNA functionalized QDs for intracellular telomerase activity monitoring. Sens Actuat B, 304, 127385(2020).
[50] G B Mao, C Liu, M Y Du et al. One-pot synthesis of the stable CdZnTeS quantum dots for the rapid and sensitive detection of copper-activated enzyme. Talanta, 185, 123(2018).
[51] G B Mao, Q Cai, F B Wang et al. One-step synthesis of rox-DNA functionalized CdZnTeS quantum dots for the visual detection of hydrogen peroxide and blood glucose. Anal Chem, 89, 11628(2017).
[52] G B Mao, M Y Du, X X Wang et al. Simple construction of ratiometric fluorescent probe for the detection of dopamine and tyrosinase by the naked eye. Analyst, 143, 5295(2018).
[53] N Q Zhan, G Palui, M Safi et al. Multidentate zwitterionic ligands provide compact and highly biocompatible quantum dots. J Am Chem Soc, 135, 13786(2013).
[54] S H Bhang, N Won, T J Lee et al. Hyaluronic acid-quantum dot conjugates forin vivo lymphatic vessel imaging. ACS Nano, 3, 1389(2009).
[55] T L Jennings, S G Becker-Catania, R C Triulzi et al. Reactive semiconductor nanocrystals for chemoselective biolabeling and multiplexed analysis. ACS Nano, 5, 5579(2011).
[56] M Wang, J L Xie, J Li et al. 3-aminophenyl boronic acid functionalized quantum-dot-based ratiometric fluorescence sensor for the highly sensitive detection of tyrosinase activity. ACS Sens, 5, 1634(2020).
[57] M Zhou, E Nakatani, L S Gronenberg et al. Peptide-labeled quantum dots for imaging GPCRs in whole cells and as single molecules. Bioconjug Chem, 18, 323(2007).
[58] Z C Feng, R N Ma, A Du et al. Enhanced performance of near-infrared-absorption CdSeTe quantum dot-sensitized solar cells via octa-aminopropyl polyhedral oligomeric silsesquioxane modification. Nano, 14, 1950087(2019).
[59] F Y Song, W C W Chan. Principles of conjugating quantum dots to proteins via carbodiimide chemistry. Nanotechnology, 22, 494006(2011).
[60] C W Chi, Y H Lao, Y S Li et al. A quantum dot-aptamer beacon using a DNA intercalating dye as the FRET reporter: Application to label-free thrombin detection. Biosens Bioelectron, 26, 3346(2011).
[61] C Schieber, A Bestetti, J P Lim et al. Conjugation of transferrin to azide-modified CdSe/ZnS core-shell quantum dots using cyclooctyne click chemistry. Angew Chem Int Ed, 51, 10523(2012).
[62] G B Mao, Y X Ma, G Q Wu et al. Novel method of clickable quantum dot construction for bioorthogonal labeling. Anal Chem, 93, 777(2021).
[63] A R Clapp, I L Medintz, J M Mauro et al. Fluorescence resonance energy transfer between quantum dot donors and dye-labeled protein acceptors. J Am Chem Soc, 126, 301(2004).
[64] C L Zhang, X H Ji, Y Zhang et al. One-pot synthesized aptamer-functionalized CdTe: Zn2+ quantum dots for tumor-targeted fluorescence imagingin vitro andin vivo. Anal Chem, 85, 5843(2013).
[65] D Wu, G F Song, Z Li et al. A two-dimensional molecular beacon for mRNA-activated intelligent cancer theranostics. Chem Sci, 6, 3839(2015).
[66] Y X Ma, G B Mao, W R Huang et al. Quantum dot nanobeacons for single RNA labeling and imaging. J Am Chem Soc, 141, 13454(2019).
[67] M Z Shen et al. Recent advances and perspectives of nucleic acid detection for coronavirus. J Pharm Anal, 10, 97(2020).
[68] L F Bustamante-Jaramillo, J Fingal, M L Blondot et al. Imaging of hepatitis B virus nucleic acids: Current advances and challenges. Viruses, 14, 557(2022).
[69] L Castillo-Henríquez, M Brenes-Acuña, A Castro-Rojas et al. Biosensors for the detection of bacterial and viral clinical pathogens. Sensors, 20, 6926(2020).
[70] A Lesiak, K Drzozga, J Cabaj et al. Optical sensors based on II-VI quantum dots. Nanomaterials, 9, 192(2019).
[71] X X Jiang, X J Liu, M Wu et al. Facile off-on fluorescence biosensing of human papillomavirus using DNA probe coupled with sunflower seed shells carbon dots. Microchem J, 181, 107742(2022).
[72] M Shamsipur, V Nasirian, K Mansouri et al. A highly sensitive quantum dots-DNA nanobiosensor based on fluorescence resonance energy transfer for rapid detection of nanomolar amounts of human papillomavirus 18. J Pharm Biomed Anal, 136, 140(2017).
[73] J H Kim, S Chaudhary, M Ozkan. Multicolour hybrid nanoprobes of molecular beacon conjugated quantum dots: FRET and gel electrophoresis assisted target DNA detection. Nanotechnology, 18, 195105(2007).
[74] A Samanta, Y D Zhou, S L Zou et al. Fluorescence quenching of quantum dots by gold nanoparticles: A potential long range spectroscopic ruler. Nano Lett, 14, 5052(2014).
[75] O Adegoke, M Morita, T Kato et al. Localized surface plasmon resonance-mediated fluorescence signals in plasmonic nanoparticle-quantum dot hybrids for ultrasensitive Zika virus RNA detection via hairpin hybridization assays. Biosens Bioelectron, 94, 513(2017).
[76] A Dove. Technology Feature| PCR: Thirty-five years and counting. Science, 360, 673(2018).
[77] Y X Wang, H Chen, H J Wei et al. Tetra-primer ARMS-PCR combined with dual-color fluorescent lateral flow assay for the discrimination of SARS-CoV-2 and its mutations with a handheld wireless reader. Lab Chip, 22, 1531(2022).
[78] P Kumar, D Pandya, N Singh et al. Loop-mediated isothermal amplification assay for rapid and sensitive diagnosis of tuberculosis. J Infect, 69, 607(2014).
[79] V L Fowler, D Armson, J L Gonzales et al. A highly effective reverse-transcription loop-mediated isothermal amplification (RT-LAMP) assay for the rapid detection of SARS-CoV-2 infection. J Infect, 82, 117(2021).
[80] S Y Wang, A L Qin, L Y Chau et al. Amine-functionalized quantum dots as a universal fluorescent nanoprobe for a one-step loop-mediated isothermal amplification assay with single-copy sensitivity. ACS Appl Mater Interfaces, 14, 35299(2022).
[81] J Y Dai, H F He, Z J Duan et al. Self-replicating catalyzed hairpin assembly for rapid signal amplification. Anal Chem, 89, 11971(2017).
[82] Y F Li, J W Li, Y Cao et al. A visual method for determination of hepatitis C virus RNAs based on a 3D nanocomposite prepared from graphene quantum dots. Anal Chimica Acta, 1203, 339693(2022).
[83] J Zhou, Q X Wang, C Y Zhang. Liposome-quantum dot complexes enable multiplexed detection of attomolar DNAs without target amplification. J Am Chem Soc, 135, 2056(2013).
[84] H Y Cui, W Q Song, Z J Cao et al. Simultaneous and sensitive detection of dual DNA targets via quantum dot-assembled amplification labels. Luminescence, 31, 281(2016).
[85] J J Wang, Y Liu, Z Ding et al. The exploration of quantum dot-molecular beacon based MoS2 fluorescence probing for myeloma-related Mirnas detection. Bioact Mater, 17, 360(2022).
[86] J S Chen, E B Ma, L B Harrington et al. CRISPR-Cas12a target binding unleashes indiscriminate single-stranded DNase activity. Science, 360, 436(2018).
[87] W H Zhou, L Hu, L M Ying et al. A CRISPR–Cas9-triggered strand displacement amplification method for ultrasensitive DNA detection. Nat Commun, 9, 5012(2018).
[88] J J Wang, C S Zheng, Y Z Jiang et al. One-step monitoring of multiple enterovirus 71 infection-related microRNAs using core-satellite structure of magnetic nanobeads and multicolor quantum dots. Anal Chem, 92, 830(2020).
[89] D D Kocak, C A Gersbach. From CRISPR scissors to virus sensors. Nature, 557, 168(2018).
[90] M D Bao, E Jensen, Y Chang et al. Magnetic bead-quantum dot (MB-qdot) clustered regularly interspaced short palindromic repeat assay for simple viral DNA detection. ACS Appl Mater Interfaces, 12, 43435(2020).
[91] Q Zhang, J H Li, Y Li et al. SARS-CoV-2 detection using quantum dot fluorescence immunochromatography combined with isothermal amplification and CRISPR/Cas13a. Biosens Bioelectron, 202, 113978(2022).
[92] L Gao, Q F Yang, P Wu et al. Recent advances in nanomaterial-enhanced enzyme-linked immunosorbent assays. Analyst, 145, 4069(2020).
[93] Y Liang, X L Huang, R J Yu et al. Fluorescence ELISA for sensitive detection of ochratoxin A based on glucose oxidase-mediated fluorescence quenching of CdTe QDs. Anal Chimica Acta, 936, 195(2016).
[94] Y Q Wu, L F Zeng, Y Xiong et al. Fluorescence ELISA based on glucose oxidase-mediated fluorescence quenching of quantum dots for highly sensitive detection of Hepatitis B. Talanta, 181, 258(2018).
[95] J J Zhou, M S Ren, W J Wang et al. Pomegranate-inspired silica nanotags enable sensitive dual-modal detection of rabies virus nucleoprotein. Anal Chem, 92, 8802(2020).
[96] W W Zhao, Y M Han, Y C Zhu et al. DNA labeling generates a unique amplification probe for sensitive photoelectrochemical immunoassay of HIV-1 p24 antigen. Anal Chem, 87, 5496(2015).
[97] A Jo, T H Kim, D M Kim et al. Sensitive detection of virus with broad dynamic range based on highly bright quantum dot-embedded nanoprobe and magnetic beads. J Ind Eng Chem, 90, 319(2020).
[98] F Nasrin, A D Chowdhury, K Takemura et al. Single-step detection of norovirus tuning localized surface plasmon resonance-induced optical signal between gold nanoparticles and quantum dots. Biosens Bioelectron, 122, 16(2018).
[99] S A Byrnes, T Huynh, T C Chang et al. Wash-free, digital immunoassay in polydisperse droplets. Anal Chem, 92, 3535(2020).
[100] Z Wu, T Zeng, W J Guo et al. Digital single virus immunoassay for ultrasensitive multiplex avian influenza virus detection based on fluorescent magnetic multifunctional nanospheres. ACS Appl Mater Interfaces, 11, 5762(2019).
[101] R Soleimani, C Deckers, T D Huang et al. Rapid COVID-19 antigenic tests: Usefulness of a modified method for diagnosis. J Med Virol, 93, 5655(2021).
[102] C W Wang, X S Yang, S Zheng et al. Development of an ultrasensitive fluorescent immunochromatographic assay based on multilayer quantum dot nanobead for simultaneous detection of SARS-CoV-2 antigen and influenza A virus. Sens Actuat B, 345, 130372(2021).
[103] H Han, C W Wang, X S Yang et al. Rapid field determination of SARS-CoV-2 by a colorimetric and fluorescent dual-functional lateral flow immunoassay biosensor. Sens Actuat B, 351, 130897(2022).
[104] L Chen, X W Zhang, C L Zhang et al. Dual-color fluorescence and homogeneous immunoassay for the determination of human enterovirus 71. Anal Chem, 83, 7316(2011).
[105] L Chen, X W Zhang, G H Zhou et al. Simultaneous determination of human Enterovirus 71 and Coxsackievirus B3 by dual-color quantum dots and homogeneous immunoassay. Anal Chem, 84, 3200(2012).
[106] X Y Zhang, Q Zhou, Z F Shen et al. Quantum dot incorporatedBacillus spore as nanosensor for viral infection. Biosens Bioelectron, 74, 575(2015).
[107] Z Yao, L Drecun, F Aboualizadeh et al. A homogeneous split-luciferase assay for rapid and sensitive detection of anti-SARS CoV-2 antibodies. Nat Commun, 12, 1806(2021).
[108] Y N Tang, T R Song, L Gao et al. A CRISPR-based ultrasensitive assay detects attomolar concentrations of SARS-CoV-2 antibodies in clinical samples. Nat Commun, 13, 4667(2022).