
- Photonics Research
- Vol. 9, Issue 12, 2475 (2021)
Abstract
1. INTRODUCTION
Fabrication of multifunctional optoelectronic devices, especially for the low-dimensional devices, has regarded as the rising demand of energy conservation, miniaturization, high-integration, being portable in many daily life aspects [1–8]. Because of its wide bandgap and a high exciton-binding energy (
As a typical wide-bandgap semiconductor,
To satisfy some special requirements in practical applications with regard to excellent electroluminescent and photovoltaic properties, studies on the synthesis of low-dimensional semiconductors and the construction of optoelectronic devices with bifunctional electronics are particularly important [5,47–50]. In this work, individual
Sign up for Photonics Research TOC. Get the latest issue of Photonics Research delivered right to you!Sign up now
2. EXPERIMENTAL SECTION
A. Synthesis of Individual
Single-crystal
B. Fabrication of
A heterostructured device made of an individual
3. RESULTS AND DISCUSSION
A.
As we described in the experimental section, individual
Figure 1.Characterization of the prepared
Figure 2.Characterization of the fabricated
The illustration in Fig. 2(a) is a schematic of a heterojunction LED, which is made of a
Varying the forward bias above the turn-on voltage, the electrically driven luminescence from the as-fabricated
To exploit the near-ultraviolet EL features of the as-fabricated
Compared with PL results of the
The near-ultraviolet emission characteristics of one-dimensional wired LED were studied by using a high numerical aperture microscope objective via a CCD camera (Olympus). Varying the injection current in the range of 3.0–13.0 mA, the optical microscopic EL images of blue–violet illuminating were captured, as shown in Fig. 3. From the figure, the near-ultraviolet light is mainly emitted out along both the lateral edges of the quadrilateral MW, and the EL intensity is periodically distributed around the quadrilateral microresonator. For the first time, the
Figure 3.When illuminated electrically at forward biasing condition, optical microscopic EL pictures of the as-fabricated
B.
As we described above, the fabricated
Figure 4.Characterization of ultraviolet photodetecting features of the prepared
When illuminated upon different light wavelengths, typical
To research the light wavelength selectivity of the as-fabricated
In the formula,
To assess the device performance of the presented
The linear dynamic range (LDR) of the
In the formula,
The photosensitivity of the
Figure 5.(a) Light power-dependent photoresponse under ultraviolet illumination at the time scale. The device was illuminated at the light wavelength of 360 nm, and measured at a reverse bias of
When operated under 360 nm ultraviolet illumination at
The stability of the as-constructed
Figure 6.(a)
The investigation of the photoresponse was performed, and the quantificational research on the processes of this current rise and decay is represented by the time-dependent photocurrent curve, which is fitted by the exponential equation as follows [2,29,30,39]:
In this equation,
Comparison between the Fabricated n-SnO2 MW/p-GaN Heterojunction Photodetector in This Work and Other Previously Reported Works
Photodetector | Wavelength | Responsivity | Detectivity [Jones] | EQE | UV/vis Ratio | Response Time | Refs. |
---|---|---|---|---|---|---|---|
320 nm | – | – | – | – | [ | ||
290 nm | 23 mA/W | – | 10% | 200 | 128/91 ms | [ | |
260 nm | – | [ | |||||
320 nm | – | – | – | – | [ | ||
370 nm | 0.36 mA/W | – | 1.8 | 0.72/1.78 s | [ | ||
300 nm | – | – | – | – | 32.2/7.8 s | [ | |
450 nm | 0.018 mA/W | – | – | – | [ | ||
300 nm | 1.6 mA/W | 0.63% | 500 | 0.045/1.17 ms | [ | ||
360 nm | 185 mA/W | 74% | 310 ns/61 μs | [ | |||
320 nm | 1900 mA/W (3 V) | – | – | 0.03/1.94 ms (0 V) | [ | ||
360 nm | 1450 mA/W | 497% | 9.2/51 ms | This work |
The working principle of the
4. CONCLUSIONS
In conclusion, we demonstrate the realization of monolithically integrated ultraviolet LED and photodetector utilizing the same
References
[1] Y. Wu, Z. Li, K.-W. Ang, Y. Jia, Z. Shi, Z. Huang, W. Yu, X. Sun, X. Liu, D. Li. Monolithic integration of MoS2-based visible detectors and GaN-based UV detectors. Photon. Res., 7, 1127-1133(2019).
[2] L. Su, Y. Zuo, J. Xie. Scalable manufacture of vertical p-GaN/n-SnO2 heterostructure for self-powered ultraviolet photodetector, solar cell and dual-color light emitting diode. InfoMat, 3, 598-610(2021).
[3] Q. Liu, Q. Xue, Y. Wang, X. Wei, J. Hao. Bifunctional device with high-energy storage density and ultralow current analog resistive switching. Adv. Electron. Mater., 7, 2000902(2021).
[4] C. Han, C. Li, Z. Zang, M. Wang, K. Sun, X. Tang, J. Du. Tunable luminescent CsPb2Br5 nanoplatelets: applications in light-emitting diodes and photodetectors. Photon. Res., 5, 473-480(2017).
[5] Q. Shan, C. Wei, Y. Jiang, J. Song, Y. Zou, L. Xu, T. Fang, T. Wang, Y. Dong, J. Liu, B. Han, F. Zhang, J. Chen, Y. Wang, H. Zeng. Perovskite light-emitting/detecting bifunctional fibres for wearable LiFi communication. Light Sci. Appl., 9, 163(2020).
[6] J. Xie, P. Hang, H. Wang, S. Zhao, G. Li, Y. Fang, F. Liu, X. Guo, H. Zhu, X. Lu, X. Yu, C. C. S. Chan, K. S. Wong, D. Yang, J. Xu, K. Yan. Perovskite bifunctional device with improved electroluminescent and photovoltaic performance through interfacial energy-band engineering. Adv. Mater., 31, 1902543(2019).
[7] W. Song, J. Chen, Z. Li, X. Fang. Self-powered MXene/GaN van der Waals heterojunction ultraviolet photodiodes with superhigh efficiency and stable current outputs. Adv. Mater., 33, 2101059(2021).
[8] Q. Cai, H. You, H. Guo, J. Wang, B. Liu, Z. Xie, D. Chen, H. Lu, Y. Zheng, R. Zhang. Progress on AlGaN-based solar-blind ultraviolet photodetectors and focal plane arrays. Light: Sci. Appl., 10, 94(2021).
[9] C. Wu, B. Du, W. Luo, Y. Liu, T. Li, D. Wang, X. Guo, H. Ting, Z. Fang, S. Wang, Z. Chen, Y. Chen, L. Xiao. Highly efficient and stable self-powered ultraviolet and deep-blue photodetector based on Cs2AgBiBr6/SnO2 heterojunction. Adv. Opt. Mater., 6, 1800811(2018).
[10] K. Liu, M. Sakurai, M. Aono. Controlling semiconducting and insulating states of SnO2 reversibly by stress and voltage. ACS Nano, 6, 7209-7215(2012).
[11] M. Periyasamy, A. Kar. Modulating the properties of SnO2 nanocrystals: morphological effects on structural, photoluminescence, photocatalytic, electrochemical and gas sensing properties. J. Mater. Chem. C, 8, 4604-4635(2020).
[12] L. Hu, J. Yan, M. Liao, L. Wu, X. Fang. Ultrahigh external quantum efficiency from thin SnO2 nanowire ultraviolet photodetectors. Small, 7, 1012-1017(2011).
[13] Y. Chen, W. Qiu, X. Wang, W. Liu, J. Wang, G. Dai, Y. Yuan, Y. Gao, J. Sun. Solar-blind SnO2 nanowire photo-synapses for associative learning and coincidence detection. Nano Energy, 62, 393-400(2019).
[14] Y. Li, W. Yin, R. Deng, R. Chen, J. Chen, Q. Yan, B. Yao, H. Sun, S.-H. Wei, T. Wu. Realizing a SnO2-based ultraviolet light-emitting diode via breaking the dipole-forbidden rule. NPG Asia Mater., 4, e30(2012).
[15] S. Pan, W. Lu, Z. Chu, G. Li. Deep ultraviolet emission from water-soluble SnO2 quantum dots grown via a facile ‘top-down’ strategy. J. Mater. Sci. Technol., 31, 670-673(2015).
[16] X. Xue, L. Zhang, X. Geng, Y. Huang, B. Zhang, Y. Zhao, M. Xu, J. Yan, D. Zhang, F. Zhao. Effect of the aln interlayer on electroluminescent performance of n-SnO2/p-GaN heterojunction light-emitting diodes. Mater. Sci. Semicond. Process., 91, 409-413(2019).
[17] H. Zhou, R. Deng, Y.-F. Li, B. Yao, Z.-H. Ding, Q.-X. Wang, Y. Han, T. Wu, L. Liu. Wavelength-tuned light emission via modifying the band edge symmetry: doped SnO2 as an example. J. Phys. Chem. C, 118, 6365-6371(2014).
[18] S. Li, S. Wang, K. Liu, N. Zhang, Z. Zhong, H. Long, G. Fang. Self-powered blue-sensitive photodetector based on PEDOT:PSS/SnO2 microwires organic/inorganic p-n heterojunction. Appl. Phys. A, 119, 1561-1566(2015).
[19] A. D. Pramata, K. Suematsu, A. T. Quitain, M. Sasaki, T. Kida. Synthesis of highly luminescent SnO2 nanocrystals: analysis of their defect-related photoluminescence using polyoxometalates as quenchers. Adv. Funct. Mater., 28, 1704620(2018).
[20] N. Bhardwaj, B. Satpati, S. Mohapatra. Plasmon-enhanced photoluminescence from SnO2 nanostructures decorated with Au nanoparticles. Appl. Surf. Sci., 504, 144381(2020).
[21] C. Lin, Y. Lu, Y. Tian, C. Gao, M. Fan, X. Yang, L. Dong, C. Shan. Diamond based photodetectors for solar-blind communication. Opt. Express, 27, 29962-29971(2019).
[22] Z. Zhang, C. Lin, X. Yang, Y. Tian, C. Gao, K. Li, J. Zang, X. Yang, L. Dong, C. Shan. Solar-blind imaging based on 2-inch polycrystalline diamond photodetector linear array. Carbon, 173, 427-432(2021).
[23] S. Li, D. Guo, P. Li, X. Wang, Y. Wang, Z. Yan, Z. Liu, Y. Zhi, Y. Huang, Z. Wu, W. Tang. Ultrasensitive, superhigh signal-to-noise ratio, self-powered solar-blind photodetector based on n-Ga2O3/p-CuSCN core-shell microwire heterojunction. ACS Appl. Mater. Interfaces, 11, 35105-35114(2019).
[24] P. Wan, M. Jiang, T. Xu, Y. Liu, C. Kan. High-mobility induced high-performance self-powered ultraviolet photodetector based on single ZnO microwire/PEDOT:PSS heterojunction via slight Ga-doping. J. Mater. Sci. Technol., 93, 33-40(2021).
[25] L. Su, W. Ouyang, X. Fang. Facile fabrication of heterostructure with p-BiOCl nanoflakes and n-ZnO thin film for UV photodetectors. J. Semicond., 42, 052301(2021).
[26] C.-N. Lin, Y.-J. Lu, X. Yang, Y.-Z. Tian, C.-J. Gao, J.-L. Sun, L. Dong, F. Zhong, W.-D. Hu, C.-X. Shan. Diamond-based all-carbon photodetectors for solar-blind imaging. Adv. Opt. Mater., 6, 1800068(2018).
[27] W. Dai, W. Liu, J. Yang, C. Xu, A. Alabastri, C. Liu, P. Nordlander, Z. Guan, H. Xu. Giant photothermoelectric effect in silicon nanoribbon photodetectors. Light Sci. Appl., 9, 120(2020).
[28] M. Zervos, N. Lathiotakis, N. Kelaidis, A. Othonos, E. Tanasa, E. Vasile. Epitaxial highly ordered Sb:SnO2 nanowires grown by the vapor liquid solid mechanism on m-, r- and a-Al2O3. Nanoscale Adv., 1, 1980-1990(2019).
[29] J. Cai, X. Xu, L. Su, W. Yang, H. Chen, Y. Zhang, X. Fang. Self-powered n-SnO2/p-CuZnS core-shell microwire UV photodetector with optimized performance. Adv. Opt. Mater., 6, 1800213(2018).
[30] Y. Zhang, W. Xu, X. Xu, J. Cai, W. Yang, X. Fang. Self-powered dual-color UV-green photodetectors based on SnO2 millimeter wire and microwires/CsPbBr3 particle heterojunctions. J. Phys. Chem. Lett., 10, 836-841(2019).
[31] L. Li, W. Gao, H. Chen, K. Zhao, P. Wen, Y. Yang, X. Wang, Z. Wei, N. Huo, J. Li. Strong anisotropy and piezo-phototronic effect in SnO2 microwires. Adv. Electron. Mater., 6, 1901441(2020).
[32] Z. Long, X. Xu, W. Yang, M. Hu, D. V. Shtansky, D. Golberg, X. Fang. Cross-bar SnO2-NiO nanofiber-array-based transparent photodetectors with high detectivity. Adv. Electron. Mater., 6, 1901048(2020).
[33] J. Yan, Y. Chen, X. Wang, Y. Fu, J. Wang, J. Sun, G. Dai, S. Tao, Y. Gao. High-performance solar-blind SnO2 nanowire photodetectors assembled using optical tweezers. Nanoscale, 11, 2162-2169(2019).
[34] C.-H. Lin, R.-S. Chen, T.-T. Chen, H.-Y. Chen, Y.-F. Chen, K.-H. Chen, L.-C. Chen. High photocurrent gain in SnO2 nanowires. Appl. Phys. Lett., 93, 112115(2008).
[35] H. Chen, L. Hu, X. Fang, L. Wu. General fabrication of monolayer SnO2 nanonets for high-performance ultraviolet photodetectors. Adv. Funct. Mater., 22, 1229-1235(2012).
[36] Q.-M. Fu, J.-L. Peng, Z.-C. Yao, H.-Y. Zhao, Z.-B. Ma, H. Tao, Y.-F. Tu, Y. Tian, D. Zhou, Y.-B. Han. Highly sensitive ultraviolet photodetectors based on ZnO/SnO2 core-shell nanorod arrays. Appl. Surf. Sci., 527, 146923(2020).
[37] W. Tian, T. Zhai, C. Zhang, S.-L. Li, X. Wang, F. Liu, D. Liu, X. Cai, K. Tsukagoshi, D. Golberg, Y. Bando. Low-cost fully transparent ultraviolet photodetectors based on electrospun ZnO-SnO2 heterojunction nanofibers. Adv. Mater., 25, 4625-4630(2013).
[38] K. Liu, M. Sakurai, M. Aono. One-step fabrication of Ga2O3-amorphous-SnO2 core-shell microribbons and their thermally switchable humidity sensing properties. J. Mater. Chem., 22, 12882-12887(2012).
[39] J. Han, M. He, M. Yang, Q. Han, F. Wang, F. Zhong, M. Xu, Q. Li, H. Zhu, C. Shan, W. Hu, X. Chen, X. Wang, J. Gou, Z. Wu, J. Wang. Light-modulated vertical heterojunction phototransistors with distinct logical photocurrents. Light Sci. Appl., 9, 167(2020).
[40] J. Zhao, R. Deng, J. Qin, J. Song, D. Jiang, B. Yao, Y. Li. Photoresponse enhancement in SnO2-based ultraviolet photodetectors via coupling with surface plasmons of Ag particles. J. Alloy. Compd., 748, 398-403(2018).
[41] K. Liu, M. Sakurai, M. Aono, D. Shen. Ultrahigh-gain single SnO2 microrod photoconductor on flexible substrate with fast recovery speed. Adv. Funct. Mater., 25, 3157-3163(2015).
[42] X. Xu, J. Chen, S. Cai, Z. Long, Y. Zhang, L. Su, S. He, C. Tang, P. Liu, H. Peng, X. Fang. A real-time wearable UV-radiation monitor based on a high-performance p-CuZnS/n-TiO2 photodetector. Adv. Mater., 30, 1803165(2018).
[43] H. Shi, B. Cheng, Q. Cai, X. Su, Y. Xiao, S. Lei. Surface state controlled ultrahigh selectivity and sensitivity for UV photodetectors based on individual SnO2 nanowires. J. Mater. Chem. C, 4, 8399-8406(2016).
[44] H. Wu, Z. Su, F. Jin, H. Zhao, W. Li, B. Chu. Improved performance of perovskite photodetectors based on a solution-processed CH3NH3PbI3/SnO2 heterojunction. Org. Electron., 57, 206-210(2018).
[45] X. Huang, Y.-Q. Yu, J. Xia, H. Fan, L. Wang, M.-G. Willinger, X.-P. Yang, Y. Jiang, T.-R. Zhang, X.-M. Meng. Ultraviolet photodetectors with high photosensitivity based on type-II ZnS/SnO2 core/shell heterostructured ribbons. Nanoscale, 7, 5311-5319(2015).
[46] L. Gan, M. Liao, H. Li, Y. Ma, T. Zhai. Geometry-induced high performance ultraviolet photodetectors in kinked SnO2 nanowires. J. Mater. Chem. C, 3, 8300-8306(2015).
[47] C. Ling, T. Guo, W. Lu, Y. Xiong, L. Zhu, Q. Xue. Ultrahigh broadband photoresponse of SnO2 nanoparticle thin film/SiO2/p-Si heterojunction. Nanoscale, 9, 8848-8857(2017).
[48] C. Jia, Z. Lin, Y. Huang, X. Duan. Nanowire electronics: from nanoscale to macroscale. Chem. Rev., 119, 9074-9135(2019).
[49] S. Chang, Y. Zhao, J. Tang, Z. Bai, L. Zhao, H. Zhong. Balanced carrier injection and charge separation of CuInS2 quantum dots for bifunctional light-emitting and photodetection devices. J. Phys. Chem. C, 124, 6554-6561(2020).
[50] S. Shrivastava, T. Q. Trung, N.-E. Lee. Recent progress, challenges, and prospects of fully integrated mobile and wearable point-of-care testing systems for self-testing. Chem. Soc. Rev., 49, 1812-1866(2020).
[51] M. Jiang, K. Tang, P. Wan, T. Xu, H. Xu, C. Kan. A single microwire near-infrared exciton-polariton light-emitting diode. Nanoscale, 13, 1663-1672(2021).
[52] M. Jiang, P. Wan, K. Tang, M. Liu, C. Kan. An electrically driven whispering gallery polariton microlaser. Nanoscale, 13, 5448-5459(2021).
[53] C. Kan, Y. Wu, J. Xu, P. Wan, M. Jiang. Plasmon-enhanced strong exciton-polariton coupling in single microwire-based heterojunction light-emitting diodes. Opt. Express, 29, 1023-1036(2021).
[54] L. Hu, J. Yan, M. Liao, H. Xiang, X. Gong, L. Zhang, X. Fang. An optimized ultraviolet-A light photodetector with wide-range photoresponse based on ZnS/ZnO biaxial nanobelt. Adv. Mater., 24, 2305-2309(2012).
[55] L. Zhang, P. Wan, T. Xu, C. Kan, M. Jiang. Flexible ultraviolet photodetector based on single ZnO microwire/polyaniline heterojunctions. Opt. Express, 29, 19202-19213(2021).
[56] Y. Li, Z. Shi, W. Liang, L. Wang, S. Li, F. Zhang, Z. Ma, Y. Wang, Y. Tian, D. Wu, X. Li, Y. Zhang, C. Shan, X. Fang. Highly stable and spectrum-selective ultraviolet photodetectors based on lead-free copper-based perovskites. Mater. Horiz., 7, 530-540(2020).
[57] Y. Zhang, S. Li, Z. Li, H. Liu, X. Liu, J. Chen, X. Fang. High-performance two-dimensional perovskite Ca2Nb3O10 UV photodetectors. Nano Lett., 21, 382-388(2021).
[58] D. Wang, X. Liu, S. Fang, C. Huang, Y. Kang, H. Yu, Z. Liu, H. Zhang, R. Long, Y. Xiong, Y. Lin, Y. Yue, B. Ge, T. K. Ng, B. S. Ooi, Z. Mi, J.-H. He, H. Sun. Pt/AlGaN nanoarchitecture: toward high responsivity, self-powered ultraviolet-sensitive photodetection. Nano Lett., 21, 120-129(2021).
[59] C. Li, H. Wang, F. Wang, T. Li, L. Shen. Ultrafast and broadband photodetectors based on a perovskite/organic bulk heterojunction for large-dynamic-range imaging. Light Sci. Appl., 9, 31(2020).
[60] T. Oshima, T. Okuno, S. Fujita. UV-B sensor based on a SnO2 thin film. Jpn. J. Appl. Phys., 48, 120207(2009).
[61] P. Chetri, J. C. Dhar. Self-powered UV detection using SnO2 nanowire arrays with Au Schottky contact. Mater. Sci. Semicond. Process., 100, 123-129(2019).
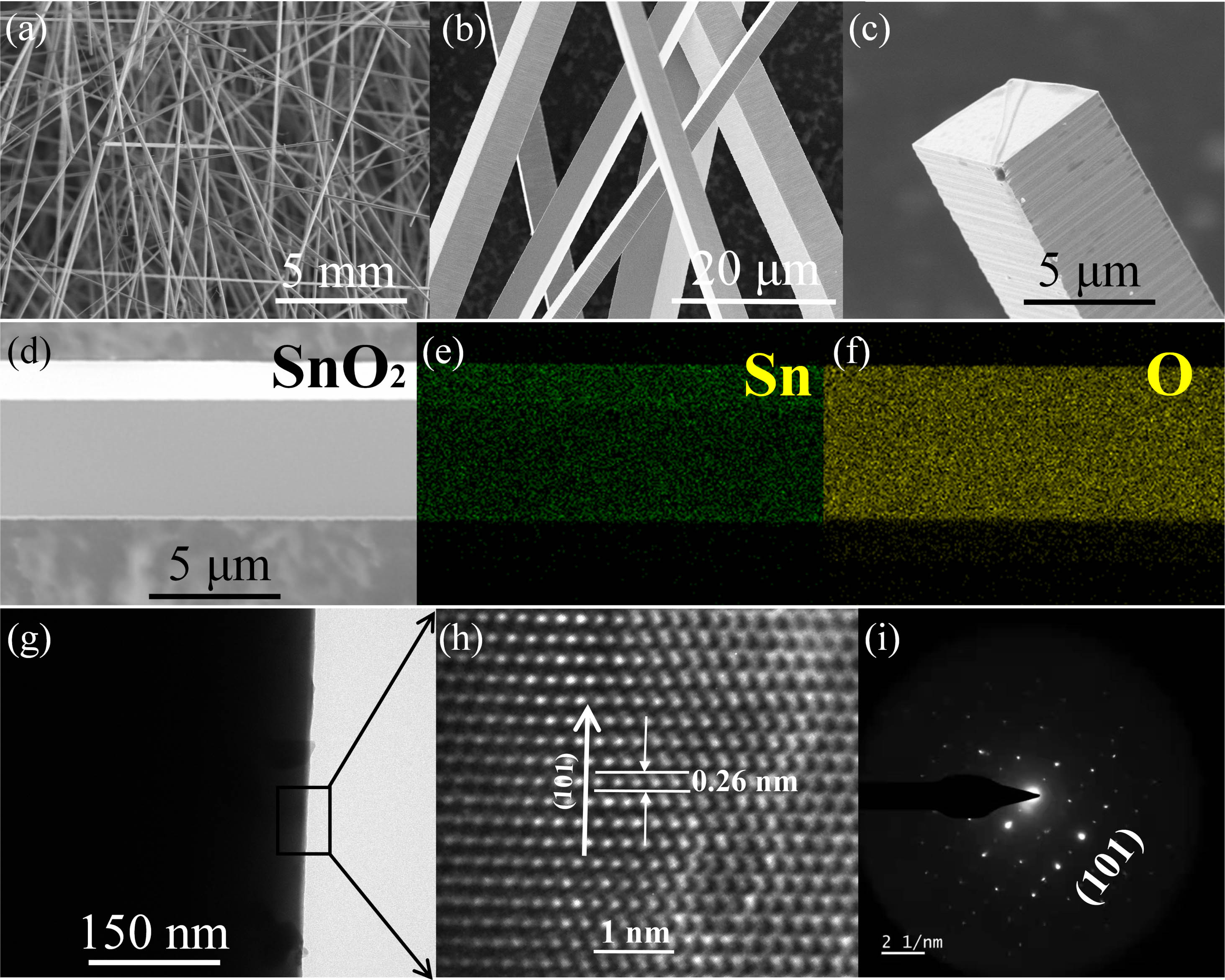
Set citation alerts for the article
Please enter your email address