Jiaye Wu, Ze Tao Xie, Yanhua Sha, H. Y. Fu, Qian Li, "Epsilon-near-zero photonics: infinite potentials," Photonics Res. 9, 1616 (2021)

Search by keywords or author
- Photonics Research
- Vol. 9, Issue 8, 1616 (2021)
![(a) Measured complex permittivity of ENZ ITO nanolayer. The ENZ wavelength is observed at 1400 nm [108]. (b) Field intensity enhancement factor versus incident angle for multilayer, CdO:Dy, and ITO [124]. (c) Schematic diagram of a waveguide structure with an ENZ section [125]. (d) Photograph of an ITO nanolayer deposited on a silica glass substrate. (e) Square quantum well model of the MIM structure with thick metal layers [126]. (f) Double barrier system of the quantum well model for MIM structure with thin metal layers [126]. (a) Springer Nature and Changchun Institute of Optics, Fine Machines and Physics (CIOMP), under a Creative Commons CC BY License; (b), (c) American Physical Society; (e), (f) American Chemical Society.](/richHtml/prj/2021/9/8/08001616/img_001.jpg)
Fig. 1. (a) Measured complex permittivity of ENZ ITO nanolayer. The ENZ wavelength is observed at 1400 nm [108]. (b) Field intensity enhancement factor versus incident angle for multilayer, CdO:Dy, and ITO [124]. (c) Schematic diagram of a waveguide structure with an ENZ section [125]. (d) Photograph of an ITO nanolayer deposited on a silica glass substrate. (e) Square quantum well model of the MIM structure with thick metal layers [126]. (f) Double barrier system of the quantum well model for MIM structure with thin metal layers [126]. (a) Springer Nature and Changchun Institute of Optics, Fine Machines and Physics (CIOMP), under a Creative Commons CC BY License; (b), (c) American Physical Society; (e), (f) American Chemical Society.
![(a) AFM scanning of F:CdO thin film [159]. (b) Scanning electron microscopy (SEM) image of the cross section of the multilayer structure [170]. (c) SEM image of the nanodisks [170]. (d) Photograph of the all-dielectric PC design and setup [21]. (e) SEM image of the deep-etched 4H-SiC gratings [171]. (a) American Chemical Society; (b), (c) De Gruyter, under a CC BY Creative Commons Attribution 4.0 International License; (d) Springer-Nature, under a Creative Commons CC BY License; (e) American Chemical Society.](/richHtml/prj/2021/9/8/08001616/img_002.jpg)
Fig. 2. (a) AFM scanning of F:CdO thin film [159]. (b) Scanning electron microscopy (SEM) image of the cross section of the multilayer structure [170]. (c) SEM image of the nanodisks [170]. (d) Photograph of the all-dielectric PC design and setup [21]. (e) SEM image of the deep-etched 4H-SiC gratings [171]. (a) American Chemical Society; (b), (c) De Gruyter, under a CC BY Creative Commons Attribution 4.0 International License; (d) Springer-Nature, under a Creative Commons CC BY License; (e) American Chemical Society.
![Calculated (a)-(d) first- to fourth-order chromatic dispersions in the ENZ region of AZO, GZO, and ITO by symbolic calculation. The values of dispersions do not decline with the increment of the order for the Drude-like permittivity ENZ materials [35]. (a)–(d) American Physical Society.](/Images/icon/loading.gif)
Fig. 3. Calculated (a)-(d) first- to fourth-order chromatic dispersions in the ENZ region of AZO, GZO, and ITO by symbolic calculation. The values of dispersions do not decline with the increment of the order for the Drude-like permittivity ENZ materials [35]. (a)–(d) American Physical Society.
![Influence of optical structure on light-matter interactions [203]. (a) Effective index dispersion of the fundamental mode for ENZ waveguides with different core sizes wx. (b) Electric E field and magnetic H field distribution at the cutoff frequency on xy plane. (c) y component of the E field along the waveguide axis at the ENZ wavelength of 870 nm and a shorter wavelength of 600 nm. Influence of the substrate [170]: phase distribution profiles of the x component of E field for ENZ nanodisk diameters of 120 nm (left) and 180 nm (right) (d) on glass at the wavelength of 684 nm; (e) on hyperbolic metamaterial at 684 nm; (f) on glass at 751 nm; and (g) on hyperbolic metamaterial at 751 nm. (a)–(c) American Physical Society, under a Creative Commons Attribution 4.0 International License; (d)–(g) De Gruyter, under a Creative Commons Attribution 4.0 International License.](/Images/icon/loading.gif)
Fig. 4. Influence of optical structure on light-matter interactions [203]. (a) Effective index dispersion of the fundamental mode for ENZ waveguides with different core sizes w x . (b) Electric E field and magnetic H field distribution at the cutoff frequency on x y plane. (c) y component of the E field along the waveguide axis at the ENZ wavelength of 870 nm and a shorter wavelength of 600 nm. Influence of the substrate [170]: phase distribution profiles of the x component of E field for ENZ nanodisk diameters of 120 nm (left) and 180 nm (right) (d) on glass at the wavelength of 684 nm; (e) on hyperbolic metamaterial at 684 nm; (f) on glass at 751 nm; and (g) on hyperbolic metamaterial at 751 nm. (a)–(c) American Physical Society, under a Creative Commons Attribution 4.0 International License; (d)–(g) De Gruyter, under a Creative Commons Attribution 4.0 International License.
![(a) AFS in ENZ materials with Drude-like dispersion [52]. (b) Refractive-index-change-defined spatial boundary and a refractive-index-change-defined boundary defined by time-refraction [53]. (c) Temporal change in refractive index and light intensity [53]. (d) Redshift or blueshift of the frequency of the probe when the pump beam falls behind or leads the probe [53]. (a) Optical Society of America. (b)–(d) Springer-Nature, under a Creative Commons CC BY License.](/Images/icon/loading.gif)
Fig. 5. (a) AFS in ENZ materials with Drude-like dispersion [52]. (b) Refractive-index-change-defined spatial boundary and a refractive-index-change-defined boundary defined by time-refraction [53]. (c) Temporal change in refractive index and light intensity [53]. (d) Redshift or blueshift of the frequency of the probe when the pump beam falls behind or leads the probe [53]. (a) Optical Society of America. (b)–(d) Springer-Nature, under a Creative Commons CC BY License.
![Schematic diagram of (a) intraband nonlinearity of ENZ materials: pump-induced permittivity change, which in turn alters the reflectivity; (b) electron effective mass modification (elevation) by pump energy absorption (absorptive loss); (c) effective-mass-change-induced plasma frequency redshift [218]. (a)–(c) Optical Society of America.](/Images/icon/loading.gif)
Fig. 6. Schematic diagram of (a) intraband nonlinearity of ENZ materials: pump-induced permittivity change, which in turn alters the reflectivity; (b) electron effective mass modification (elevation) by pump energy absorption (absorptive loss); (c) effective-mass-change-induced plasma frequency redshift [218]. (a)–(c) Optical Society of America.
![(a) Relation between complex permittivity of ENZ ITO and free carrier concentration [80]. (b) Controlled annealing protocol (temperature) for ITO for ENZ wavelength tuning [68]. (c) Temperature, dopant ratio, and thickness dependence of ENZ AZO in ALD fabrication and condition control [224]. (a) IEEE, under a Creative Commons Attribution 4.0 License; (b) AIP Publishing; (c) John Wiley and Sons.](/Images/icon/loading.gif)
Fig. 7. (a) Relation between complex permittivity of ENZ ITO and free carrier concentration [80]. (b) Controlled annealing protocol (temperature) for ITO for ENZ wavelength tuning [68]. (c) Temperature, dopant ratio, and thickness dependence of ENZ AZO in ALD fabrication and condition control [224]. (a) IEEE, under a Creative Commons Attribution 4.0 License; (b) AIP Publishing; (c) John Wiley and Sons.
![Schematic diagrams of (a) multilayered metal-MO stack isolator [98]; (b) waveguide-based isolator [98]; (c) non-Hermitian ENZ optical isolation scheme [237]. (a), (b) Optical Society of America; (c) John Wiley and Sons.](/Images/icon/loading.gif)
Fig. 8. Schematic diagrams of (a) multilayered metal-MO stack isolator [98]; (b) waveguide-based isolator [98]; (c) non-Hermitian ENZ optical isolation scheme [237]. (a), (b) Optical Society of America; (c) John Wiley and Sons.
![(a) Layout of the Q-switched waveguide using the ENZ ITO [238]. (b) Reflectance spectra of CdO-based Bragg microcavity under different pump fluences at a 45° incident angle [76]. (c) Transmission of the all-optical switch under different pump light intensities at 30° incident angle; the inset is the schematic of the all-optical switch [65]. (d) Schematic of the Si photonic waveguide, and transmission of the device in ON and OFF states [240]. (e) Schematic of the MIM nanocavity with two ENZ modes. The high energy (HE) and low energy (LE) resonances display low reflectance at the ENZ points (top). Change of R for the signal probe pulse upon optical pumping of the HE ENZ mode; the gray curve represents the equilibrium positions of the resonances [79]. (f) Schematic of the dielectric guided-mode resonance gratings. The reflectance of the three primary color devices at the color-on state (no bias) and color-off state (under bias). CIE 1931 coordinates calculated by the proposed device (solid line) and the color gamut of the sRGB triangle (dashed line) [241]. (a) De Gruyter, under a Creative Commons Attribution 4.0 International License; (b) Optical Society of America; (c) IEEE, under a Creative Commons Attribution 4.0 International License; (d) Chinese Laser Press; (e) Spring-Nature, under a Creative Commons Attribution 4.0 International License; (f) Royal Society of Chemistry.](/Images/icon/loading.gif)
Fig. 9. (a) Layout of the Q -switched waveguide using the ENZ ITO [238]. (b) Reflectance spectra of CdO-based Bragg microcavity under different pump fluences at a 45° incident angle [76]. (c) Transmission of the all-optical switch under different pump light intensities at 30° incident angle; the inset is the schematic of the all-optical switch [65]. (d) Schematic of the Si photonic waveguide, and transmission of the device in ON and OFF states [240]. (e) Schematic of the MIM nanocavity with two ENZ modes. The high energy (HE) and low energy (LE) resonances display low reflectance at the ENZ points (top). Change of R for the signal probe pulse upon optical pumping of the HE ENZ mode; the gray curve represents the equilibrium positions of the resonances [79]. (f) Schematic of the dielectric guided-mode resonance gratings. The reflectance of the three primary color devices at the color-on state (no bias) and color-off state (under bias). CIE 1931 coordinates calculated by the proposed device (solid line) and the color gamut of the sRGB triangle (dashed line) [241]. (a) De Gruyter, under a Creative Commons Attribution 4.0 International License; (b) Optical Society of America; (c) IEEE, under a Creative Commons Attribution 4.0 International License; (d) Chinese Laser Press; (e) Spring-Nature, under a Creative Commons Attribution 4.0 International License; (f) Royal Society of Chemistry.
![(a) Schematic of the chip-scale electro-absorption modulator and SEM image of fabrication result [84]. (b) Schematic and SEM image of the gigahertz speed ENZ modulator, and transmission of the modulator under DC electro-optical characterization [64]. (c) Schematic and SEM image of coupling-enhanced dual-gated ITO modulator, cross-sectional image, and mode profile illustrating the device layers and operating mechanism of the active region [252]. (d) Schematic of the Mach-Zehnder modulator; Si/ITO/Al2O3/Au active device region, and corresponding eigenmode profiles to light ON and OFF states; optical microscope image of the Mach-Zehnder modulator [253]. (a) American Chemical Society; (b) Optical Society of America; (c) De Gruyter, under a Creative Commons Attribution 4.0 International License; (d) Optical Society of America.](/Images/icon/loading.gif)
Fig. 10. (a) Schematic of the chip-scale electro-absorption modulator and SEM image of fabrication result [84]. (b) Schematic and SEM image of the gigahertz speed ENZ modulator, and transmission of the modulator under DC electro-optical characterization [64]. (c) Schematic and SEM image of coupling-enhanced dual-gated ITO modulator, cross-sectional image, and mode profile illustrating the device layers and operating mechanism of the active region [252]. (d) Schematic of the Mach-Zehnder modulator; Si / ITO / Al 2 O 3 / Au active device region, and corresponding eigenmode profiles to light ON and OFF states; optical microscope image of the Mach-Zehnder modulator [253]. (a) American Chemical Society; (b) Optical Society of America; (c) De Gruyter, under a Creative Commons Attribution 4.0 International License; (d) Optical Society of America.
![(a) Illustrations of an electrically tunable absorber, and the reflectance spectra measured using a Fourier-transform IR microscope. The red, blue, and green lines represent the reflectance of the device under 0 V for no bias, −5 V for accumulation, and +5 V for depletion, respectively; the gray curves represent reflectance with an incremental step size of 1 V [90]. (b) SEM image of the fabricated device and experimentally measured absorption spectrum compared with simulated absorption spectrum [274]. (c) SEM images from the fabricated Ti3C2Tx disk array on glass, Ti3C2Tx disk array on Au/alumina, and experimentally measured absorption spectra for transverse-electric (TE) and TM polarizations for two designs [278]. (a) Springer-Nature, under a Creative Commons CC BY License; (b), (c) American Chemical Society.](/Images/icon/loading.gif)
Fig. 11. (a) Illustrations of an electrically tunable absorber, and the reflectance spectra measured using a Fourier-transform IR microscope. The red, blue, and green lines represent the reflectance of the device under 0 V for no bias, − 5 V for accumulation, and + 5 V for depletion, respectively; the gray curves represent reflectance with an incremental step size of 1 V [90]. (b) SEM image of the fabricated device and experimentally measured absorption spectrum compared with simulated absorption spectrum [274]. (c) SEM images from the fabricated Ti 3 C 2 T x disk array on glass, Ti 3 C 2 T x disk array on Au/alumina, and experimentally measured absorption spectra for transverse-electric (TE) and TM polarizations for two designs [278]. (a) Springer-Nature, under a Creative Commons CC BY License; (b), (c) American Chemical Society.
![(a) Sketch of ENZ bodies, numerical prediction radiation pattern based on the normalized emission pattern at the ENZ wavelength [71]. (b) Thermal emission around embedded eigenstate [292]. (a) National Academy of Sciences; (b) American Physical Society.](/Images/icon/loading.gif)
Fig. 12. (a) Sketch of ENZ bodies, numerical prediction radiation pattern based on the normalized emission pattern at the ENZ wavelength [71]. (b) Thermal emission around embedded eigenstate [292]. (a) National Academy of Sciences; (b) American Physical Society.
|
Table 1. Comparison of ENZ Electro-Optical Modulatorsa
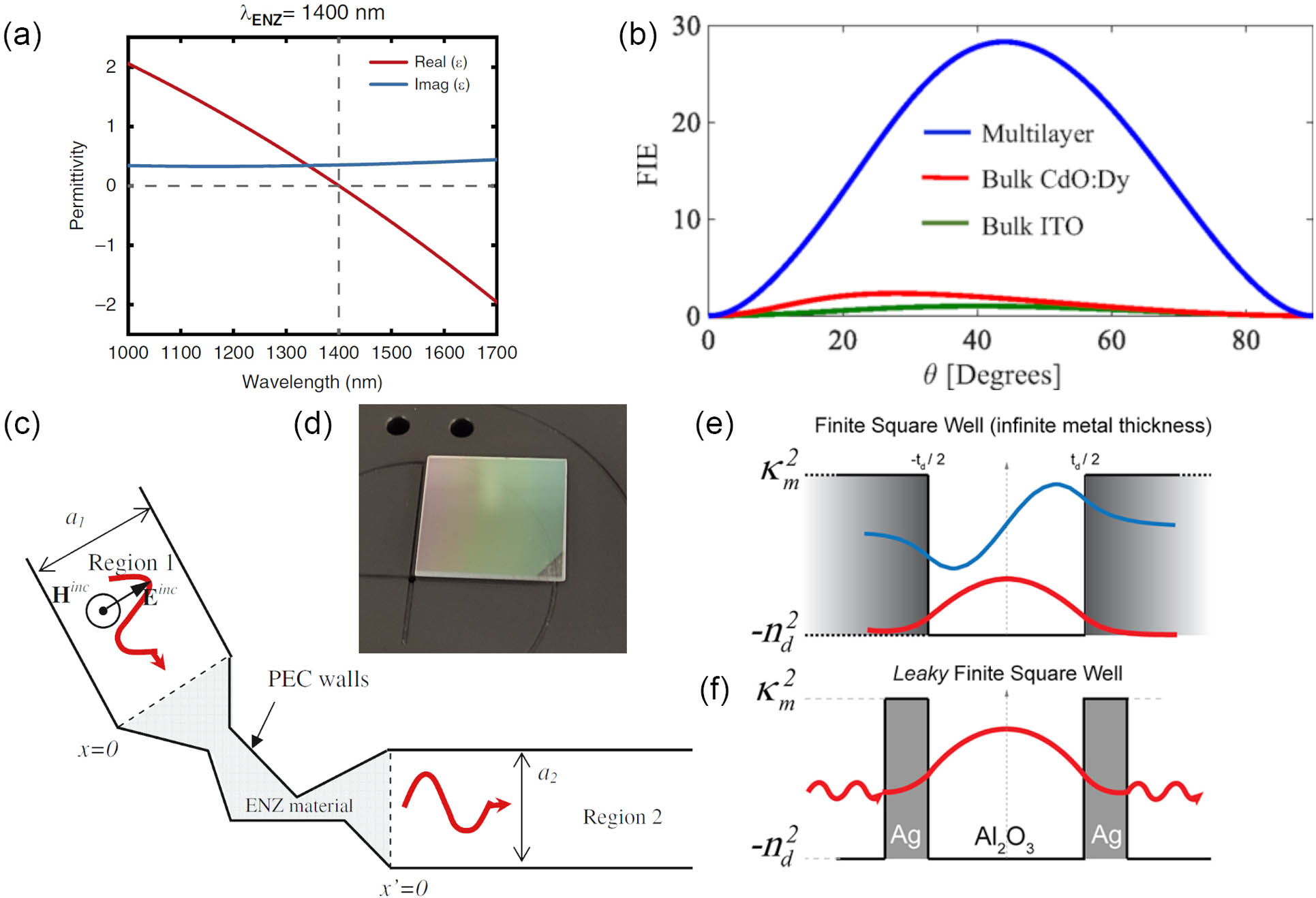
Set citation alerts for the article
Please enter your email address