
- Photonics Research
- Vol. 10, Issue 5, 1290 (2022)
Abstract
1. INTRODUCTION
On-chip optical frequency combs could revolutionize a vast range of applications such as optical atomic clocks [1], astronomy [2,3], coherent optical communication [4,5], ultrafast distance measurements by means of LIDAR [6–9], spectroscopy [10], and integrated microwave photonics [11,12]. It could also be applied in optical neural computing [13,14] and quantum optics [15,16]. Silicon nitride (
A summary of progress in
Figure 1.Overview of the propagation loss in low-temperature
As an alternative, low-temperature (
Sign up for Photonics Research TOC. Get the latest issue of Photonics Research delivered right to you!Sign up now
Several groups invested much effort in reducing the propagation loss of low-temperature
In addition to the removal of the hydrogen bond, a key factor in improving the quality of the
In this paper, we report the first-known experimental generation of such soliton frequency combs in a high-
2. CHARACTERISTICS OF THE LOW-TEMPERATURE ICP-CVD
All
Figure 2.(a) Measured and fitted
The dependence of propagation loss on the wavelength shown in Fig. 2(b) is obtained by fitting 454 fundamental transverse electric (
3. GENERATION OF SOLITON FREQUENCY COMBS
A 160-μm-radius MRR shown in Fig. 3(a) with the same waveguide cross section and bus waveguide coupling as the previous device is selected to conduct the Kerr frequency comb experiment. The measured FSR is around 150 GHz, and fiber-chip coupling loss is 2.35 dB per facet with the use of inverse taper mode expanders at either end of the bus waveguide. As shown in Fig. 3(b), resonances of the
Figure 3.(a) Micrograph of a
In order to readily access and study dissipative Kerr solitons (DKSs) in the
The high
The single soliton state with the repetition rate of 150 GHz is further characterized because its symmetric and smooth
Multiple MRR devices with different radii were fabricated on the same
Figure 4.Optical spectra of single soliton combs with repetition rates of 50–240 GHz generated from MRRs with different radii. The enlarged insets show the spectral details from 1519 to 1522 nm wavelength range.
4. EXPERIMENTAL SECTION
A. Device Fabrication
The
Devices in this work are fabricated solely by subtractive processing. The pattern of MRR devices is defined by means of electron beam lithography (EBL) in AR-P 6200 resist with a thickness of 800 nm and then is transferred to the
B. Device Characterization
The transmission spectra of MRRs are measured by wavelength scanning using a Keysight 8164B Lightwave Measurement System. The output from a narrow-linewidth tunable laser (Keysight 81606A TLS) is edge-coupled through a single-mode lensed fiber to the inverse-tapered
C. Soliton Frequency Comb Generation Setup
An auxiliary laser is used to mitigate the thermo-optical effects to allow the robust generation of DKSs in
5. CONCLUSION
By means of an optimized ICP-CVD process using deuterated silane (
High
References
[1] Z. L. Newman, V. Maurice, T. Drake, J. R. Stone, T. C. Briles, D. T. Spencer, C. Fredrick, Q. Li, D. Westly, B. R. Ilic, B. Shen, M.-G. Suh, K. Y. Yang, C. Johnson, D. M. S. Johnson, L. Hollberg, K. J. Vahala, K. Srinivasan, S. A. Diddams, J. Kitching, S. B. Papp, M. T. Hummon. Architecture for the photonic integration of an optical atomic clock. Optica, 6, 680-685(2019).
[2] E. Obrzud, M. Rainer, A. Harutyunyan, M. H. Anderson, J. Liu, M. Geiselmann, B. Chazelas, S. Kundermann, S. Lecomte, M. Cecconi, A. Ghedina, E. Molinari, F. Pepe, F. Wildi, F. Bouchy, T. J. Kippenberg, T. Herr. A microphotonic astrocomb. Nat. Photonics, 13, 31-35(2019).
[3] M. G. Suh, X. Yi, Y. H. Lai, S. Leifer, I. S. Grudinin, G. Vasisht, E. C. Martin, M. P. Fitzgerald, G. Doppmann, J. Wang, D. Mawet, S. B. Papp, S. A. Diddams, C. Beichman, K. Vahala. Searching for exoplanets using a microresonator astrocomb. Nat. Photonics, 13, 25-30(2019).
[4] P. Marin-Palomo, J. N. Kemal, M. Karpov, A. Kordts, J. Pfeifle, M. H. P. Pfeiffer, P. Trocha, S. Wolf, V. Brasch, M. H. Anderson, R. Rosenberger, K. Vijayan, W. Freude, T. J. Kippenberg, C. Koos. Microresonator-based solitons for massively parallel coherent optical communications. Nature, 546, 274-279(2017).
[5] A. Fülöp, M. Mazur, A. Lorences-Riesgo, Ó. B. Helgason, P. H. Wang, Y. Xuan, D. E. Leaird, M. Qi, P. A. Andrekson, A. M. Weiner, V. Torres-Company. High-order coherent communications using mode-locked dark-pulse Kerr combs from microresonators. Nat. Commun., 9, 1(2018).
[6] M. G. Suh, K. J. Vahala. Soliton microcomb range measurement. Science, 359, 884-887(2018).
[7] P. Trocha, M. Karpov, D. Ganin, M. H. P. Pfeiffer, A. Kordts, S. Wolf, J. Krockenberger, P. Marin-Palomo, C. Weimann, S. Randel, W. Freude, T. J. Kippenberg, C. Koos. Ultrafast optical ranging using microresonator soliton frequency combs. Science, 359, 887-891(2018).
[8] J. Riemensberger, A. Lukashchuk, M. Karpov, W. Weng, E. Lucas, J. Liu, T. J. Kippenberg. Massively parallel coherent laser ranging using a soliton microcomb. Nature, 581, 164-170(2020).
[9] J. Wang, Z. Lu, W. Wang, F. Zhang, J. Chen, Y. Wang, J. Zheng, S. T. Chu, W. Zhao, B. E. Little, X. Qu, W. Zhang. Long-distance ranging with high precision using a soliton microcomb. Photon. Res., 8, 1964-1972(2020).
[10] M. G. Suh, Q. F. Yang, K. Y. Yang, X. Yi, K. J. Vahala. Microresonator soliton dual-comb spectroscopy. Science, 354, 600-603(2016).
[11] W. Liang, D. Eliyahu, V. S. Ilchenko, A. A. Savchenkov, A. B. Matsko, D. Seidel, L. Maleki. High spectral purity Kerr frequency comb radio frequency photonic oscillator. Nat. Commun., 6, 7957(2015).
[12] D. T. Spencer, S. H. Lee, D. Y. Oh, M.-G. Suh, K. Y. Yang, K. Vahala. An optical-frequency synthesizer using integrated photonics. Nature, 557, 81-85(2018).
[13] X. Xu, M. Tan, B. Corcoran, J. Wu, A. Boes, T. G. Nguyen, S. T. Chu, B. E. Little, D. G. Hicks, R. Morandotti, A. Mitchell, D. J. Moss. 11 TOPS photonic convolutional accelerator for optical neural networks. Nature, 589, 44-51(2021).
[14] J. Feldmann, N. Youngblood, M. Karpov, H. Gehring, X. Li, M. Stappers, M. Le Gallo, X. Fu, A. Lukashchuk, A. S. Raja, J. Liu, C. D. Wright, A. Sebastian, T. J. Kippenberg, W. H. P. Pernice, H. Bhaskaran. Parallel convolutional processing using an integrated photonic tensor core. Nature, 589, 52-58(2021).
[15] Z. Yang, M. Jahanbozorgi, D. Jeong, S. Sun, O. Pfister, H. Lee, X. Yi. A squeezed quantum microcomb on a chip. Nat. Commun., 12, 1(2021).
[16] Y. Zhang, M. Menotti, K. Tan, V. D. Vaidya, D. H. Mahler, L. G. Helt, L. Zatti, M. Liscidini, B. Morrison, Z. Vernon. Squeezed light from a nanophotonic molecule. Nat. Commun., 12, 8(2021).
[17] M. W. Puckett, K. Liu, N. Chauhan, Q. Zhao, N. Jin, H. Cheng, J. Wu, R. O. Behunin, P. T. Rakich, K. D. Nelson, D. J. Blumenthal. 422 million intrinsic quality factor planar integrated all-waveguide resonator with sub-MHz linewidth. Nat. Commun., 12, 934(2021).
[18] P. Del’Haye, A. Schliesser, O. Arcizet, T. Wilken, R. Holzwarth, T. J. Kippenberg. Optical frequency comb generation from a monolithic microresonator. Nature, 450, 1214-1217(2007).
[19] T. Herr, V. Brasch, J. D. Jost, C. Y. Wang, N. M. Kondratiev, M. L. Gorodetsky, T. J. Kippenberg. Temporal solitons in optical microresonators. Nat. Photonics, 8, 145-152(2014).
[20] X. Xue, Y. Xuan, Y. Liu, P. H. Wang, S. Chen, J. Wang, D. E. Leaird, M. Qi, A. M. Weiner. Mode-locked dark pulse Kerr combs in normal-dispersion microresonators. Nat. Photonics, 9, 594-600(2015).
[21] D. C. Cole, E. S. Lamb, P. Del’Haye, S. A. Diddams, S. B. Papp. Soliton crystals in Kerr resonators. Nat. Photonics, 11, 671-676(2017).
[22] J. Chiles, N. Nader, D. D. Hickstein, S. P. Yu, T. C. Briles, D. Carlson, H. Jung, J. M. Shainline, S. Diddams, S. B. Papp, S. W. Nam, R. P. Mirin. Deuterated silicon nitride photonic devices for broadband optical frequency comb generation. Opt. Lett., 43, 1527-1530(2018).
[23] Z. Wu, Y. Zhang, S. Zeng, J. Li, Y. Xie, Y. Chen, S. Yu. Low-noise Kerr frequency comb generation with low temperature deuterated silicon nitride waveguides. Opt. Express, 29, 29557-29566(2021).
[24] C. Xiang, J. Liu, J. Guo, L. Chang, R. N. Wang, W. Weng, J. Peters, W. Xie, Z. Zhang, J. Riemensberger, J. Selvidge, T. J. Kippenberg, J. E. Bowers. Laser soliton microcombs heterogeneously integrated on silicon. Science, 373, 99-103(2021).
[25] Z. Wu, Z. Shao, Z. Xu, Y. Zhang, L. Liu, C. Yang, Y. Chen, S. Yu. High quality factor deuterated silicon nitride (SiN:D) microring resonators. Conference on Lasers and Electro-Optics/Pacific Rim, W4D.5(2018).
[26] A. Frigg, A. Boes, G. Ren, I. Abdo, D.-Y. Choi, S. Gees, A. Mitchell. Low loss CMOS-compatible silicon nitride photonics utilizing reactive sputtered thin films. Opt. Express, 27, 37795-37805(2019).
[27] H. El Dirani, A. Kamel, M. Casale, S. Kerdiles, C. Monat, X. Letartre, M. Pu, L. K. Oxenløwe, H. El Dirani, A. Kamel, M. Casale, S. Kerdiles, C. Monat, H. El Dirani, A. Kamel, M. Casale, S. Kerdiles. Annealing-free Si3N4 frequency combs for monolithic integration with Si photonics. Appl. Phys. Lett., 113, 081102(2018).
[28] T. Hiraki, T. Aihara, H. Nishi, T. Tsuchizawa. Deuterated SiN/SiON waveguides on Si platform and their application to C-band WDM filters. IEEE Photon. J., 9, 2500207(2017).
[29] A. V. Osinsky, R. A. Bellman, I. A. Akwani, P. A. Sachenik, S. L. Logunov, J. W. McCamy. Optical loss mechanisms in GeSiON planar waveguides. Appl. Phys. Lett., 81, 2002-2004(2002).
[30] Z. Wu, S. Zeng, Y. Chen, Y. Zhang, S. Yu. Frequency comb generation in a deuterated-SiN
[31] S. Tang, Y. Zhang, Z. Wu, L. Zhou, L. Liu, Y. Chen, Y. Yu. Tunable microwave photonic filter based on silicon nitride MZI-assist micro-ring resonator. Asia Communications and Photonics Conference, M3E.4(2019).
[32] T. Hiraki, T. Aihara, K. Takeda, T. Fujii, T. Kakitsuka, T. Tsuchizawa, H. Fukuda, S. Matsuo. Membrane InGaAsP Mach-Zehnder modulator with SiN:D waveguides on Si platform. Opt. Express, 27, 18612-18619(2019).
[33] H. Nishi, T. Fuji, N. P. Diamantopoulos, K. Takeda, E. Kanno, T. Kakitsuka, T. Tsuchizawa, H. Fukuda, S. Matsuo. Integration of eight-channel directly modulated membrane-laser array and SiN AWG multiplexer on Si. J. Lightwave Technol., 37, 266-273(2019).
[34] Z. Wu, Z. Xu, Y. Zhang, H. Chen, Y. Chen, S. Yu. Four-wave mixing parametric oscillation in deuterated silicon nitride microresonators prepared by low-temperature (100 °C) PECVD platform. European Conference on Lasers and Electro-Optics, ce_4_5(2019).
[35] Z. Wu, Y. Chen, Z. Xu, L. Liu, H. Chen, Y. Zhang, S. Yu. Waveguide-integrated deuterated silicon nitride (SiN:D) microdisk resonators for nonlinear photonics. Asia Communications and Photonics Conference, 1-3(2019).
[36] D. T. Spencer, J. F. Bauters, M. J. R. Heck, J. E. Bowers. Integrated waveguide coupled Si3N4 in the ultrahigh-
[37] W. Jin, Q. F. Yang, L. Chang, B. Shen, H. Wang, M. A. Leal, L. Wu, M. Gao, A. Feshali, M. Paniccia, K. J. Vahala, J. E. Bowers. Hertz-linewidth semiconductor lasers using CMOS-ready ultra-high-
[38] K. Luke, A. Dutt, C. B. Poitras, M. Lipson. Overcoming Si3N4 film stress limitations for high quality factor ring resonators. Opt. Express, 21, 22829-22833(2013).
[39] Q. Li, A. A. Eftekhar, M. Sodagar, Z. Xia, A. H. Atabaki, A. Adibi. Vertical integration of high-
[40] M. H. P. Pfeiffer, J. Liu, A. S. Raja, T. Morais, B. Ghadiani, T. J. Kippenberg. Ultra-smooth silicon nitride waveguides based on the Damascene reflow process: fabrication and loss origins. Optica, 5, 884-892(2018).
[41] H. El Dirani, L. Youssef, C. Petit-Etienne, S. Kerdiles, P. Grosse, C. Monat, E. Pargon, C. Sciancalepore. Ultralow-loss tightly confining Si3N4 waveguides and high-
[42] Z. Ye, K. Twayana, P. A. Andrekson, V. Torres-Company. High-
[43] X. Ji, J. K. Jang, U. D. Dave, M. Corato-Zanarella, C. Joshi, A. L. Gaeta, M. Lipson. Exploiting ultralow loss multimode waveguides for broadband frequency combs. Laser Photon. Rev., 15, 2000353(2020).
[44] J. Liu, G. Huang, R. N. Wang, J. He, A. S. Raja, T. Liu, N. J. Engelsen, T. J. Kippenberg. High-yield, wafer-scale fabrication of ultralow-loss, dispersion-engineered silicon nitride photonic circuits. Nat. Commun., 12, 2236(2021).
[45] W. Jin, D. D. John, J. F. Bauters, T. Bosch, B. J. Thibeault, J. E. Bowers. Deuterated silicon dioxide for heterogeneous integration of ultra-low-loss waveguides. Opt. Lett., 45, 3340-3343(2020).
[46] X. Ji, F. A. S. Barbosa, S. P. Roberts, A. Dutt, J. Cardenas, Y. Okawachi, A. Bryant, A. L. Gaeta, M. Lipson. Ultra-low-loss on-chip resonators with sub-milliwatt parametric oscillation threshold. Optica, 4, 619-624(2017).
[47] H. Zhou, Y. Geng, W. Cui, S. W. Huang, Q. Zhou, K. Qiu, C. Wei Wong. Soliton bursts and deterministic dissipative Kerr soliton generation in auxiliary-assisted microcavities. Light Sci. Appl., 8, 1(2019).
[48] T. Herr, K. Hartinger, J. Riemensberger, C. Y. Wang, E. Gavartin, R. Holzwarth, M. L. Gorodetsky, T. J. Kippenberg. Universal formation dynamics and noise of Kerr-frequency combs in microresonators. Nat. Photonics, 6, 480-487(2012).
[49] M. Karpov, H. Guo, A. Kordts, V. Brasch, M. H. P. Pfeiffer, M. Zervas, M. Geiselmann, T. J. Kippenberg. Raman self-frequency shift of dissipative Kerr solitons in an optical microresonator. Phys. Rev. Lett., 116, 103902(2016).
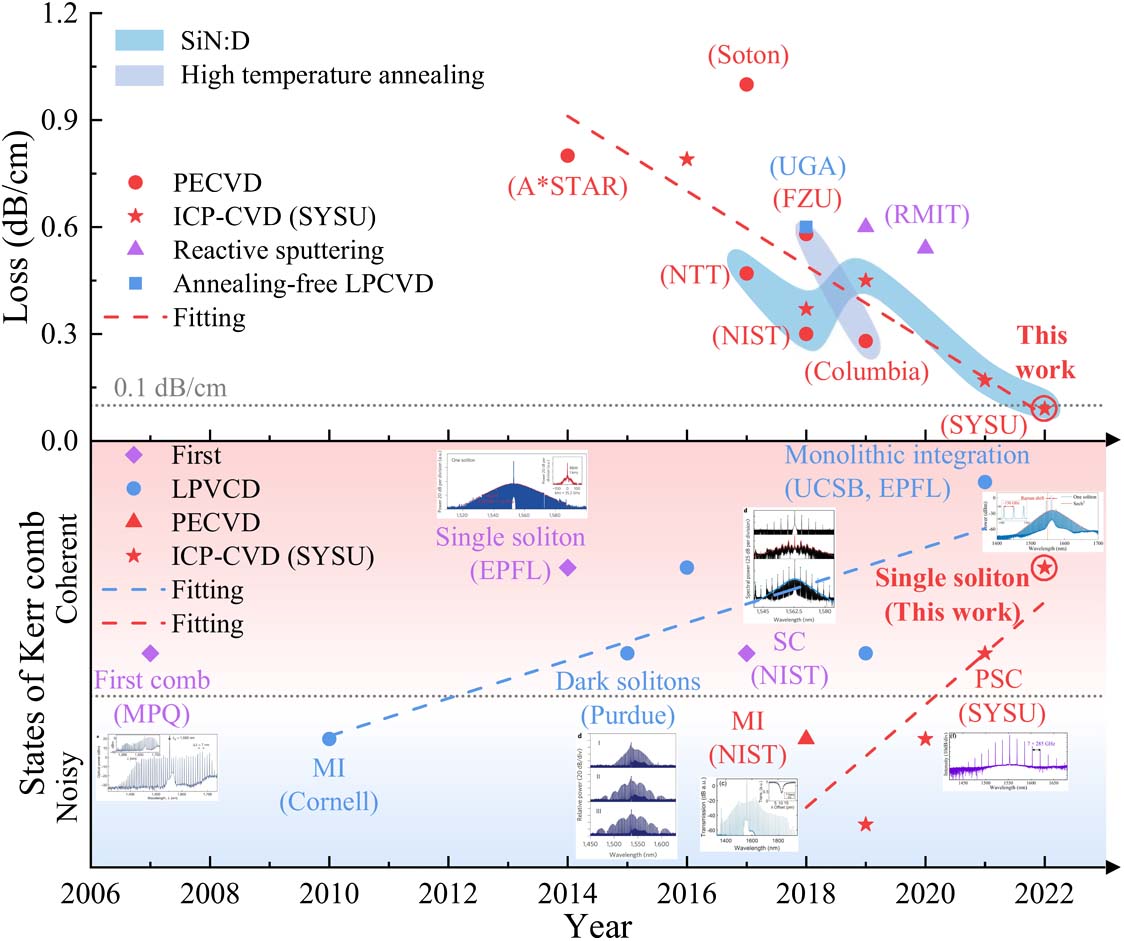
Set citation alerts for the article
Please enter your email address