
- Chinese Optics Letters
- Vol. 19, Issue 10, 100101 (2021)
Abstract
1. Introduction
Since 1992, the vortex beam has been proved to possess orbital angular momentum (OAM)[
However, scattering is always a fundamental limit in optical applications. A beam of coherent light incident into a turbid medium will be scattered and produce the speckle of random intensity. Over the past few years, the propagation and penetration properties of optical vortex beams in the turbid medium have been investigated[
The feedback-based wavefront shaping (FBWS) method was proposed by Vellekoop and Mosk in 2007[
Sign up for Chinese Optics Letters TOC. Get the latest issue of Chinese Optics Letters delivered right to you!Sign up now
In this Letter, assisted by the FBWS method, we restore the OAM information of light behind strongly scattering media. By using an SPP with an opposite topological charge as a detection component, the objective intensity steadily increases and approaches the local optimum with the operation of the genetic evolution algorithm. The enhancement factor is estimated to be 150 over 500 generations of the evolution. These experimental results are repeatable and suitable for OAM recovery of different topological charges. In addition, the reconstitution of OAMs around different optical axes is also demonstrated in our experiment.
2. Experiment
The concept of reconstituting optical OAMs via the FBWS method is shown in Fig. 1. Without wavefront shaping, the light behind the scattered material is mutilated as disordered speckles on the screen, as shown in Fig. 1(a). According to Huygens’ principle, each point of the original spiral wavefront emits a wavelet. The optical pattern is the result of propagation and coherent superposition of every wavelet. When going through the strongly scattering medium, each wavelet gets a random additional phase (as well as attenuation), which leads to the generation of the disordered speckle pattern. Such OAM states of light can be reconstituted forwardly by using a wavefront optimization procedure after the strongly scattering media, as shown in Fig. 1(b). In the reconstruction processes, the SPP with opposite topological charge is used to detect the relative intensity of light carrying the specific OAM[
Figure 1.Sketch map of optical OAM reconstitution through scattering media. (a) Without wavefront shaping, the OAM beam forms a disordered speckle pattern behind the scattering medium. (b) With an appropriate SLM phase mask applied in advance, optical OAM is reconstituted after scattering. Using an SPP with an opposite topological charge as a detection component, a focal point can be generated on the screen.
The experimental setup is illustrated in Fig. 2. A continuous wave (CW) laser at the wavelength of 532 nm is used as the light source. A half-wave plate (HWP) and a polarizer (
Figure 2.Experimental setup for OAM restoration behind the strongly scattering media. HWP, half-wavelength plate;
Before algorithm optimization, it is important to set a suitable objective function for iteration. As mentioned above, the detection system is formed by an SPP, a far-field diffractive lens, and a CMOS camera, which forms the Fourier transform relationship in the plane of these three optical components. Firstly, the system is calibrated without the scattering medium. Figure 3 shows an experimental demonstration of measuring optical OAM. The intensity graph in Fig. 3(a) is the OAM state with
Figure 3.Experimental demonstration of measuring OAM and calibration. (a) An example for the OAM state with
3. Results
The complete reconstitution process of an OAM state of
Figure 4.(a) Calibration of detecting system with an SPP of
Figure 5.(a) Enhancement factor curves of different topological charges (ℓ = 4–7). In our experiment, a typical value is 150 after 500 generations. (b) Stability measurement of the focusing relative intensity over 10 h.
As we know, the definition of OAM of light is determined by the direction of the optical axis. Besides, the manipulation of OAM at different directions also brings flexibility when it is used in different circumstances. Therefore, based on the same system, we also restore the OAM of light at different spatial directions in our experiment. By adjusting the angle of the reflect mirror after the SLM, the optical axis orientation of the light path can be adjusted in a small range. The calibration and optimization processes are similar to the previous. Figure 6 shows the experiments on the reconstitution of the OAM beam with
Figure 6.Reconstitution of OAMs in different spatial directions. (a) and (b) have a deflection angle of around
4. Discussion
The main limit of the enhancement factor in our experiment comes from the modulation depth and degree of freedom of the SLM. In theory, more input modes will result in a higher enhancement[
In conclusion, we have reported the reconstitution of optical OAMs scattered by strongly turbid media via the FBWS method. In our experiment, the SPP with an opposite topological charge is used to detect the OAM of scattered light. Using algorithms to address random scattering problems effectively avoids mathematically complex operations. Combining the optimization algorithm, the optical OAM information can be effectively extracted and enhanced after the strong scattering process. The enhancement factor was estimated to be 150 after optimization of 500 generations. Meanwhile, we have verified the feasibility of this method for OAM reconstitution of different topological charges and different spatial directions. This method has been confirmed to have good repeatability and robustness. Our system is also proven to have high stability. Actually, the enhancement of the scattered OAM states is a localized effect in a particular direction by wavelet path selection and constructive interference. Nevertheless, it still demonstrates both a unique perspective and significant progress in random optics. Consequently, this work paves a way for the use of OAM beams in complex media for optical communication and deep imaging.
References
[1] L. Allen, M. W. Beijersbergen, R. J. Spreeuw, J. P. Woerdman. Orbital angular momentum of light and the transformation of Laguerre–Gaussian laser modes. Phys. Rev. A, 45, 8185(1992).
[2] S. S. Oemrawsingh, J. A. van Houwelingen, E. R. Eliel, J. P. Woerdman, E. J. Verstegen, J. G. Kloosterboer, G. W. 't Hooft. Production and characterization of spiral phase plates for optical wavelengths. Appl. Opt., 43, 688(2004).
[3] N. R. Heckenberg, R. McDuff, C. P. Smith, A. G. White. Generation of optical phase singularities by computer-generated holograms. Opt. Lett., 17, 221(1992).
[4] A. M. Yao, M. J. Padgett. Orbital angular momentum: origins, behavior and applications. Adv. Opt. Photon., 3, 161(2011).
[5] J. Arlt, K. Dholakia, L. Allen, M. J. Padgett. The production of multiringed Laguerre–Gaussian modes by computer-generated holograms. J. Mod. Opt., 45, 1231(1998).
[6] E. Karimi, B. Piccirillo, E. Nagali, L. Marrucci, E. Santamato. Efficient generation and sorting of orbital angular momentum eigenmodes of light by thermally tuned q-plates. Appl. Phys. Lett., 94, 231124(2009).
[7] G. C. G. Berkhout, M. W. Beijersbergen. Method for probing the orbital angular momentum of optical vortices in electromagnetic waves from astronomical objects. Phys. Rev. Lett., 101, 100801(2008).
[8] G. C. Berkhout, M. P. Lavery, J. Courtial, M. W. Beijersbergen, M. J. Padgett. Efficient sorting of orbital angular momentum states of light. Phys. Rev. Lett., 105, 153601(2010).
[9] Y. Wang, X. Ma, M. Pu, X. Li, C. Huang, W. Pan, B. Zhao, J. Cui, X. Luo. Transfer of orbital angular momentum through sub-wavelength waveguides. Opt. Express, 23, 2857(2015).
[10] N. Bozinovic, Y. Yue, Y. Ren, M. Tur, P. Kristensen, H. Huang, A. E. Willner, S. Ramachandran. Terabit-scale orbital angular momentum mode division multiplexing in fibers. Science, 340, 1545(2013).
[11] Z. Liu, S. Yan, H. Liu, X. Chen. Superhigh-resolution recognition of optical vortex modes assisted by a deep-learning method. Phys. Rev. Lett., 123, 183902(2019).
[12] N. B. Simpson, L. Allen, M. J. Padgett. Optical tweezers and optical spanners with Laguerre–Gaussian modes. J. Mod. Opt., 43, 2485(1996).
[13] H. He, M. E. Friese, N. R. Heckenberg, H. Rubinsztein-Dunlop. Direct observation of transfer of angular momentum to absorptive particles from a laser beam with a phase singularity. Phys. Rev. Lett., 75, 826(1995).
[14] K. Ladavac, D. G. Grier. Microoptomechanical pumps assembled and driven by holographic optical vortex arrays. Opt. Express, 12, 1144(2004).
[15] M. P. J. Lavery, F. C. Speirits, S. M. Barnett, M. J. Padgett. Detection of a spinning object using light’s orbital angular momentum. Science, 341, 537(2013).
[16] A. Mair, A. Vaziri, G. Weihs, A. Zeilinger. Entanglement of the orbital angular momentum states of photons. Nature, 412, 313(2001).
[17] J. T. Barreiro, T.-C. Wei, P. G. Kwiat. Beating the channel capacity limit for linear photonic superdense coding. Nat. Phys., 4, 282(2008).
[18] F. Tamburini, G. Anzolin, G. Umbriaco, A. Bianchini, C. Barbieri. Overcoming the Rayleigh criterion limit with optical vortices. Phys. Rev. Lett., 97, 163903(2006).
[19] Z. Y. Zhou, Y. Li, D. S. Ding, W. Zhang, S. Shi, B. S. Shi. Optical vortex beam based optical fan for high-precision optical measurements and optical switching. Opt. Lett., 39, 5098(2014).
[20] V. D’Ambrosio, N. Spagnolo, L. Del Re, S. Slussarenko, Y. Li, L. C. Kwek, L. Marrucci, S. P. Walborn, L. Aolita, F. Sciarrino. Photonic polarization gears for ultra-sensitive angular measurements. Nat. Commun., 4, 2432(2013).
[21] D. Palacios, D. Rozas, G. A. Swartzlander. Observed scattering into a dark optical vortex core. Phys. Rev. Lett., 88, 103902(2002).
[22] M. Luo, Q. Chen, L. Hua, D. Zhao. Propagation of stochastic electromagnetic vortex beams through the turbulent biological tissues. Phys. Lett. A, 378, 308(2014).
[23] L. Shi, L. Lindwasser, W. Wang, R. Alfano, A. Rodriguez-Contreras. Propagation of Gaussian and Laguerre–Gaussian vortex beams through mouse brain tissue. J. Biophoton., 10, 1756(2017).
[24] W. B. Wang, R. Gozali, L. Shi, L. Lindwasser, R. R. Alfano. Deep transmission of Laguerre–Gaussian vortex beams through turbid scattering media. Opt. Lett., 41, 2069(2016).
[25] R. Fickler, M. Ginoya, R. W. Boyd. Custom-tailored spatial mode sorting by controlled random scattering. Phys. Rev. B, 95, 161108(2017).
[26] T. Y. Lin, A. Liu, X. P. Zhang, H. Li, L. P. Wang, H. L. Han, Z. Chen, X. P. Liu, H. B. Lü. Analyzing OAM mode purity in optical fibers with CNN-based deep learning. Chin. Opt. Lett., 17, 100603(2019).
[27] L. Chen, R. K. Singh, A. Dogariu, Z. Chen, J. Pu. Estimating topological charge of propagating vortex from single-shot non-imaged speckle. Chin. Opt. Lett., 19, 022603(2021).
[28] L. Gong, Q. Zhao, H. Zhang, X. Y. Hu, K. Huang, J. M. Yang, Y. M. Li. Optical orbital-angular-momentum-multiplexed data transmission under high scattering. Light Sci. Appl., 8, 27(2019).
[29] I. M. Vellekoop, A. P. Mosk. Focusing coherent light through opaque strongly scattering media. Opt. Lett., 32, 2309(2007).
[30] I. M. Vellekoop, A. P. Mosk. Phase control algorithms for focusing light through turbid media. Opt. Commun., 281, 3071(2008).
[31] A. Boniface, M. Mounaix, B. Blochet, R. Piestun, S. Gigan. Transmission-matrix-based point-spread-function engineering through a complex medium. Optica, 4, 54(2017).
[32] D. B. Conkey, A. N. Brown, A. M. Caravaca-Aguirre, R. Piestun. Genetic algorithm optimization for focusing through turbid media in noisy environments. Opt. Express, 20, 4840(2012).
[33] Y. Qiao, Y. Peng, Y. Zheng, F. Ye, X. Chen. Second-harmonic focusing by a nonlinear turbid medium via feedback-based wavefront shaping. Opt. Lett., 42, 1895(2017).
[34] A. Daniel, L. Liberman, Y. Silberberg. Wavefront shaping for glare reduction. Optica, 3, 1104(2016).
[35] M. Mounaix, D. Andreoli, H. Defienne, G. Volpe, O. Katz, S. Gresillon, S. Gigan. Spatiotemporal coherent control of light through a multiple scattering medium with the multispectral transmission matrix. Phys. Rev. Lett., 116, 253901(2016).
[36] O. Katz, P. Heidmann, M. Fink, S. Gigan. Non-invasive single-shot imaging through scattering layers and around corners via speckle correlations. Nat. Photon., 8, 784(2014).
[37] J. Bertolotti, E. G. van Putten, C. Blum, A. Lagendijk, W. L. Vos, A. P. Mosk. Non-invasive imaging through opaque scattering layers. Nature, 491, 232(2012).
[38] S. Yoon, M. Kim, M. Jang, Y. Choi, W. Choi, S. Kang, W. Choi. Deep optical imaging within complex scattering media. Nat. Rev. Phys., 2, 141(2020).
[39] R. Horstmeyer, H. Ruan, C. Yang. Guidestar-assisted wavefront-shaping methods for focusing light into biological tissue. Nat. Photon., 9, 563(2015).
[40] J. Jin, J. Luo, X. Zhang, H. Gao, X. Li, M. Pu, P. Gao, Z. Zhao, X. Luo. Generation and detection of orbital angular momentum via metasurface. Sci. Rep., 6, 24286(2016).
[41] G. Gibson, J. Courtial, M. Padgett, M. Vasnetsov, V. Pas’ko, S. Barnett, S. Franke-Arnold. Free-space information transfer using light beams carrying orbital angular momentum. Opt. Express, 12, 5448(2004).
[42] R. Corey, M. Kissner, P. Saulnier. Coherent backscattering of light. Am. J. Phys., 63, 560(1995).
[43] P. C. de Oliveira, A. E. Perkins, N. M. Lawandy. Coherent backscattering from high-gain scattering media. Opt. Lett., 21, 1685(1996).
[44] K. Nam, J. H. Park. Increasing the enhancement factor for DMD-based wavefront shaping. Opt. Lett., 45, 3381(2020).
[45] A. P. Mosk, A. Lagendijk, G. Lerosey, M. Fink. Controlling waves in space and time for imaging and focusing in complex media. Nat. Photon., 6, 283(2012).
[46] I. M. Vellekoop. Feedback-based wavefront shaping. Opt. Express, 23, 12189(2015).
[47] O. Katz, E. Small, Y. Bromberg, Y. Silberberg. Focusing and compression of ultrashort pulses through scattering media. Nat. Photon., 5, 372(2011).
[48] Y. Guan, O. Katz, E. Small, J. Zhou, Y. Silberberg. Polarization control of multiply scattered light through random media by wavefront shaping. Opt. Lett., 37, 4663(2012).
[49] E. Small, O. Katz, Y. Guan, Y. Silberberg. Spectral control of broadband light through random media by wavefront shaping. Opt. Lett., 37, 3429(2012).
[50] S. Popoff, G. Lerosey, M. Fink, A. C. Boccara, S. Gigan. Image transmission through an opaque material. Nat. Commun., 1, 81(2010).
[51] S. M. Popoff, G. Lerosey, R. Carminati, M. Fink, A. C. Boccara, S. Gigan. Measuring the transmission matrix in optics: an approach to the study and control of light propagation in disordered media. Phys. Rev. Lett., 104, 100601(2010).
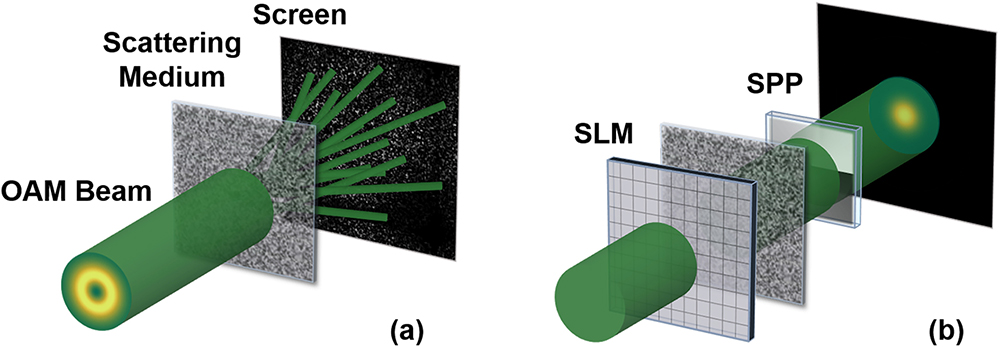
Set citation alerts for the article
Please enter your email address