
- Chinese Optics Letters
- Vol. 20, Issue 1, 013601 (2022)
Abstract
1. Introduction
Traditional bulky and heavy optical lenses are gradually difficult to keep pace with the requirements of the rapid development of miniaturized and integrated optical components/systems. However, considering the strong and unique capability of locally manipulating the amplitude, phase, and polarization to shape the wavefront of light with subwavelength resolution, planar metalenses consisting of plasmonic or dielectric nano-scatterers may play a critical role[
For the transmissive metalens working at a specific wavelength regardless of the bandwidth, its efficiency can be optimized by designing the proper geometry and dimension of meta-atoms based on the materials with low enough absorptivity to reasonably impart the target phase. A good case in point is the amorphous silicon metalens designed for an operating wavelength of 1550 nm with a high measured focusing efficiency of up to 82%[
Here, we proposed an aperture-shared partition phase cooperative manipulation (APPCM) approach to design broadband achromatic metalenses with high focusing efficiency and a narrow range of FL change. The metalens is designed into two concentric sub-metalenses with inner circular and outer ring regions for different FLs, respectively. Both the phase profiles are combined to form a wavefront of the target metalens. The achromatic polarization-independent
Sign up for Chinese Optics Letters TOC. Get the latest issue of Chinese Optics Letters delivered right to you!Sign up now
2. Methods
2.1. Modified method for broadband achromatic metalens design
The phase profile of a metalens can be derived from Fermat’s theorem[
According to Eqs. (2) and (3), the phase profiles at an arbitrary operation wavelength of
Then, the phase at other wavelengths becomes
Figure 1.Schematic diagram of the phase profiles of broadband achromatic metalenses. (a) The phase profile of a generally designed achromatic metalens. The gray dashed line is the theoretically calculated phase profile of focal length f. Due to the phase shift Δφ of the selected meta-atoms determined using a periodic boundary condition, the actual phase profile of the metalens becomes a brown solid line, and the corresponding focal length changes to f'. For other wavelengths in the bandwidth range, the focal lengths have similar changes, so that the focal lengths in the entire bandwidth range are not exactly the same, and the purpose of the wide bandwidth cannot be achieved. (b) The phase profile of a broadband achromatic metalens using APPCM. For a certain wavelength λ, the green solid line is the phase profile with focal length f1 designed in the Z1 zone (inner), the blue solid line is the phase profile with focal length f2 designed in the Z2 zone (outer), and the red solid line is the overall phase profile corresponding to the focal length f, which is between f1 and f2. The combination of both phase profiles and the compensation of the phase shifts caused by both the zones make the overall focus lengths stay almost the same for all wavelengths within the bandwidth.
Figure 2.(a) Schematic of an achromatic metalens composed of cylindrical Si3N4 ride meta-atoms. The height of the hollow nano-cylinder is 1500 nm, and the period of the meta-atoms (P) is 450 nm. (b) Wavenumber dependences of transmission (blue) and phase (red) for the meta-atom. The high transmission and a nearly linear phase as a function of the wavenumber are achieved in the entire visible region. The inset shows the geometry of the hollow nano-cylinders, where the outer and inner radii of the ring are 170 nm and 70 nm, respectively.
To address this issue, we proposed a strategy to reduce the influence of the phase shift that occurred in the general method above. We modify the method by focusing on the changes of the phase profile of the metalens composed of all meta-atoms instead of the phase shift of each meta-atom. The superposition principle of the wavefronts of light allows us to propose an APPCM approach of designing a broadband achromatic metalens, which is illustrated in Fig. 1(b). The designed metalens consists of two aperture-shared sub-metalenses Z1 and Z2. The meta-atoms in the inner circular Z1 and outer ring Z2 trim the phase profiles of
Diffraction theory is utilized to decide the position of the focal point. When a plane wave irradiates on two sub-metalenses, the output intensity
2.2. Design of the broadband achromatic metalens
As mentioned above, the entire metalens was designed into two sub-metalenses, Z1 and Z2, using APPCM. The meta-atoms in Z1 and Z2 were optimized individually by using the general method and manipulating the phase profiles of the sub-metalenses with the FLs of
The wavenumber dependences of the phase and transmittance of nano-cylinders were obtained by simulation using the finite-difference time-domain (FDTD) method[
To verify the concept of above APPCM, we designed a broadband achromatic metalens (M1) with a 950 nm bandwidth in the wavelength range of 450–1400 nm using the hollow nano-cylinder
Figure 3.(a) Simulation of the phase manipulation of Si3N4 nano-cylinders. The light blue solid dots represent the phases imparted on the nano-cylinders with different inner and outer radii at the maximum and minimum wavelengths. The black and green stars are the theoretically calculated phases for the Z1 zone (f1 = 200 µm) and the Z2 zone (f2 = 250 µm), respectively. It should be noted that some black stars and green stars overlap due to different diameters and FLs for Z1 and Z2. The first black star on the upper right and the last green star on the lower left represent the first unit and the last unit, respectively. All units are arranged in order from top to bottom to be matched. (b) Phase profiles of the broadband achromatic metalens M1 designed using the APPCM approach. The phase manipulation using the chosen nano-cylinders (red) is basically following the theoretical calculations (blue).
The phase profiles of the metalens (M1) composed of the chosen nano-cylinders versus the radius of the metalens are shown in Fig. 3(b). The phases in both zones can be found to be basically consistent with the corresponding theoretical calculations. It should be noted that the simulated phase of the selected structural unit is different from the phase of our theoretical design. We have theoretically proved that a common amount of phase shift is completely equivalent (see Section 3 of Supplementary Materials).
3. Results and Discussion
The performance of the broadband achromatic metalens designed using the APPCM approach was simulated under the illumination of a normally incident
Figure 4.Normalized intensity distribution of the broadband achromatic metalens M1. (a) Simulated normalized intensity profiles in the plane x = 0 (y–z plane) around the focal point of metalens M1 at different wavelengths. The gray dashed line is the average (214 µm) of the focal lengths for all wavelengths. (b) Intensity profiles in the focal plane (x–y plane) of M1 at different wavelengths. (c) Longitudinal cross sections of the corresponding focal spots in (b).
It is worth noting that the FL of a broadband achromatic metalens usually varies at different wavelengths due to the errors between the phase manipulation by the meta-atoms and the theoretical one. To better evaluate the discrepancies of FLs at different wavelengths, we introduced the concept of the coefficient of variation (CV) in statistics[
Here, CV for M1 is derived to be 4.05%, which is within the allowed scope (5%) of the international standard of chromatic aberration. To demonstrate that an achromatic metalens designed using the APPCM approach can improve the FL variation, we took the sub-metalens Z1 as an individual metalens with FL of 200 µm for comparison. The deviation of FLs for Z1 is shown in Fig. 5(a) (gray round-dot-line) and Supplementary Materials, Fig. S7(a). The maximum discrepancy reaches as large as 80 µm with
Figure 5.Performances of broadband achromatic metalenses M1 and M2. (a) Focal length as a function of wavelength for metalenses M1 and M2. The round-dot line and the diamond-dot line represent the focal lengths of M1 and M2, respectively. The red and blue lines are the focal lengths of the entire metalens, whereas the gray line is the focal length of the inner zone. The green and yellow shadow areas demonstrate the DOF of M1 and M2 at different wavelengths, respectively. (b) Simulated focal lengths of M1 for X, Y polarizations (X-LP, Y-LP), right-handed circular polarization (RCP), and right-handed elliptical polarization (REP). (c) Simulated FWHMs at different wavelengths. The red round and blue diamond dots are the FWHMs of M1 and M2, respectively. The green and yellow dotted lines represent the theoretical FWHMs of metalenses M1 and M2. (d) The red round and blue diamond dots give the wavelength dependence of the focusing efficiency of metalenses M1 and M2, respectively.
The nano-cylinder-based metalenses are intuitively expected to be polarization-independent[
Diffraction-limited focal spots of lenses are recognized to be capable of improving the imaging resolution, which is a key performance parameter for imaging systems[
4. Conclusion
Accurate phase manipulation for a metalens based on metasurfaces is normally indispensable for the realization of powerful functionalities with superior performances, but it is quite challenging. We thus proposed the APPCM approach, which makes it possible to design high-performance metalenses in terms of the effects of phase shift compensation and phase error correction for different wavelengths. The distortion of phase shifts would possibly lead to the fluctuation of focal length with the wavelength, the degradation of focusing efficiency, and the bandwidth narrowing of a metalens. We successfully demonstrated the feasibility of the APPCM approach by designing the high-performance metalens with the hollow nano-cylindrical
References
[1] Q. T. Li, F. Dong, B. Wang, F. Gan, J. Chen, Z. Song, L. Xu, W. Chu, Y. F. Xiao, Q. Gong, Y. Li. Polarization-independent and high-efficiency dielectric metasurfaces for visible light. Opt. Express, 24, 16309(2016).
[2] A. Arbabi, E. Arbabi, Y. Horie, S. M. Kamali, A. Faraon. Planar metasurface retroreflector. Nat. Photon., 11, 415(2017).
[3] Y. Zhou, I. I. Kravchenko, H. Wang, H. Zheng, G. Gu, J. Valentine. Multifunctional metaoptics based on bilayer metasurfaces. Light Sci. Appl., 8, 80(2019).
[4] S. Wu, Z. Zhang, Y. Zhang, K. Zhang, L. Zhou, X. Zhang, Y. Zhu. Enhanced rotation of the polarization of a light beam transmitted through a silver film with an array of perforated S-shaped holes. Phys. Rev. Lett., 110, 207401(2013).
[5] Z. Xuan, J. Li, Q. Liu, F. Yi, S. Wang, W. Lu. Artificial structural colors and applications. Innovation, 2, 100081(2021).
[6] M. Deng, T. Ren, J. Wang, L. Chen. Doublet achromatic metalens for broadband optical retroreflector. Chin. Opt. Lett., 19, 071701(2021).
[7] A. Pors, M. G. Nielsen, R. L. Eriksen, S. I. Bozhevolnyi. Broadband focusing flat mirrors based on plasmonic gradient metasurfaces. Nano Lett., 13, 829(2013).
[8] H. Yang, G. Li, X. Su, G. Cao, Z. Zhao, X. Chen, W. Lu. Reflective metalens with sub-diffraction-limited and multifunctional focusing. Sci. Rep., 7, 12632(2017).
[9] M. Khorasaninejad, F. Capasso. Metalenses: versatile multifunctional photonic components. Science, 358(2017).
[10] Z. Li, C. Cheng, H. Liu, D. Li, S. Xu, H. Liu, J. Zhang, S. Zhang. Experimental generation of Kagome lattices using metasurface of integrated convex lens. Chin. Opt. Lett., 18, 012201(2020).
[11] C. Jin, J. Zhang, C. Guo. Metasurface integrated with double-helix point spread function and metalens for three-dimensional imaging. Nanophotonics, 8, 451(2019).
[12] W.-L. Guo, G.-M. Wang, K. Chen, H.-P. Li, Y.-Q. Zhuang, H.-X. Xu, Y. Feng. Broadband polarization-conversion metasurface for a cassegrain antenna with high polarization purity. Phys. Rev. Appl., 12, 014009(2019).
[13] W. Wang, Z. Guo, K. Zhou, L. Ran, Y. Sun, F. Shen, G. Fan, Y. Li, S. Qu, S. Liu. Metalens focusing the co-/cross-polarized lights in longitudinal direction. Plasmonics, 12, 69(2016).
[14] D. Tang, C. Wang, Z. Zhao, Y. Wang, M. Pu, X. Li, P. Gao, X. Luo. Ultrabroadband superoscillatory lens composed by plasmonic metasurfaces for subdiffraction light focusing. Laser Photon. Rev., 9, 713(2015).
[15] Y. Bao, Q. Jiang, Y. Kang, X. Zhu, Z. Fang. Enhanced optical performance of multifocal metalens with conic shapes. Light Sci. Appl., 6, e17071(2017).
[16] C. Williams, Y. Montelongo, T. D. Wilkinson. Plasmonic metalens for narrowband dual-focus imaging. Adv. Opt. Mater., 5, 1700811(2017).
[17] Y. Yao, W. Wu. All-dielectric heterogeneous metasurface as an efficient ultra-broadband reflector. Adv. Opt. Mater., 5, 1700090(2017).
[18] M. Khorasaninejad, Z. Shi, A. Y. Zhu, W. T. Chen, V. Sanjeev, A. Zaidi, F. Capasso. Achromatic metalens over 60 nm bandwidth in the visible and metalens with reverse chromatic dispersion. Nano Lett., 17, 1819(2017).
[19] X. Zhang, C. Guan, K. Wang, L. Cheng, J. Yang, J. Shi, H. Liu, Z. Liu, L. Yuan. Multi-focus optical fiber lens based on all-dielectric metasurface. Chin. Opt. Lett., 19, 050601(2021).
[20] R. J. Lin, V.-C. Su, S. Wang, M. K. Chen, T. L. Chung, Y. H. Chen, H. Y. Kuo, J.-W. Chen, J. Chen, Y.-T. Huang, J.-H. Wang, C. H. Chu, P. C. Wu, T. Li, Z. Wang, S. Zhu, D. P. Tsai. Achromatic metalens array for full-colour light-field imaging. Nat. Nanotechnol., 14, 227(2019).
[21] H. Pahlevaninezhad, M. Khorasaninejad, Y. W. Huang, Z. Shi, L. P. Hariri, D. C. Adams, V. Ding, A. Zhu, C. W. Qiu, F. Capasso, M. J. Suter. Nano-optic endoscope for high-resolution optical coherence tomography in vivo. Nat. Photon., 12, 540(2018).
[22] Z.-B. Fan, H.-Y. Qiu, H.-L. Zhang, X.-N. Pang, L.-D. Zhou, L. Liu, H. Ren, Q.-H. Wang, J.-W. Dong. A broadband achromatic metalens array for integral imaging in the visible. Light Sci. Appl., 8, 67(2019).
[23] M. Khorasaninejad, A. Y. Zhu, C. Roques-Carmes, W. T. Chen, J. Oh, I. Mishra, R. C. Devlin, F. Capasso. Polarization-insensitive metalenses at visible wavelengths. Nano Lett., 16, 7229(2016).
[24] A. Arbabi, Y. Horie, A. J. Ball, M. Bagheri, A. Faraon. Subwavelength-thick lenses with high numerical apertures and large efficiency based on high-contrast transmit arrays. Nat. Commun., 6, 7069(2015).
[25] S. Wang, P. C. Wu, V. C. Su, Y. C. Lai, M. K. Chen, H. Y. Kuo, B. H. Chen, Y. H. Chen, T. T. Huang, J. H. Wang, R. M. Lin, C. H. Kuan, T. Li, Z. Wang, S. Zhu, D. P. Tsai. A broadband achromatic metalens in the visible. Nat. Nanotechnol., 13, 227(2018).
[26] W. T. Chen, A. Y. Zhu, V. Sanjeev, M. Khorasaninejad, Z. Shi, E. Lee, F. Capasso. A broadband achromatic metalens for focusing and imaging in the visible. Nat. Nanotechnol., 13, 220(2018).
[27] W. T. Chen, A. Y. Zhu, J. Sisler, Z. Bharwani, F. Capasso. A broadband achromatic polarization-insensitive metalens consisting of anisotropic nanostructures. Nat. Commun., 10, 355(2019).
[28] H. P. Zhou, L. Chen, F. Shen, K. Guo, Z. Y. Guo. Broadband achromatic metalens in the midinfrared range. Phys. Rev. Appl., 11, 024066(2019).
[29] S. Wang, P. C. Wu, V. C. Su, Y. C. Lai, C. Hung Chu, J. W. Chen, S. H. Lu, J. Chen, B. Xu, C. H. Kuan, T. Li, S. Zhu, D. P. Tsai. Broadband achromatic optical metasurface devices. Nat. Commun., 8, 187(2017).
[30] B. Yu, J. Wen, X. Chen, D. Zhang. An achromatic metalens in the near-infrared region with an array based on a single nano-rod unit. Appl. Phys. Express, 12, 092003(2019).
[31] F. Balli, M. Sultan, S. K. Lami, J. T. Hastings. A hybrid achromatic metalens. Nat. Commun., 11, 3892(2020).
[32] A. Ndao, L. Hsu, J. Ha, J. H. Park, C. Chang-Hasnain, B. Kante. Octave bandwidth photonic fishnet-achromatic-metalens. Nat. Commun., 11, 3205(2020).
[33] S. Shrestha, A. C. Overvig, M. Lu, A. Stein, N. Yu. Broadband achromatic dielectric metalenses. Light Sci. Appl., 7, 85(2018).
[34] N. Yu, P. Genevet, M. A. Kats, F. Aieta, J. P. Tetienne, F. Capasso, Z. Gaburro. Light propagation with phase discontinuities: generalized laws of reflection and refraction. Science, 334, 333(2011).
[35] L. Hsu, M. Dupre, A. Ndao, J. Yellowhair, B. Kante. Local phase method for designing and optimizing metasurface devices. Opt. Express, 25, 24974(2017).
[36] Z.-B. Fan, Z.-K. Shao, M.-Y. Xie, X.-N. Pang, W.-S. Ruan, F.-L. Zhao, Y.-J. Chen, S.-Y. Yu, J.-W. Dong. Silicon nitride metalenses for close-to-one numerical aperture and wide-angle visible imaging. Phys. Rev. Appl., 10, 014005(2018).
[37] V. Liu, S. Fan. S4: a free electromagnetic solver for layered periodic structures. Comput. Phys. Commun., 183, 2233(2012).
[38] Z. P. Zhuang, R. Chen, Z. B. Fan, X. N. Pang, J. W. Dong. High focusing efficiency in subdiffraction focusing metalens. Nanophotonics, 8, 1279(2019).
[39] G. P. Rédei, G. P. Rédei. Coefficient of variation. Encyclopedia of Genetics, Genomics, Proteomics and Informatics, 385(2008).
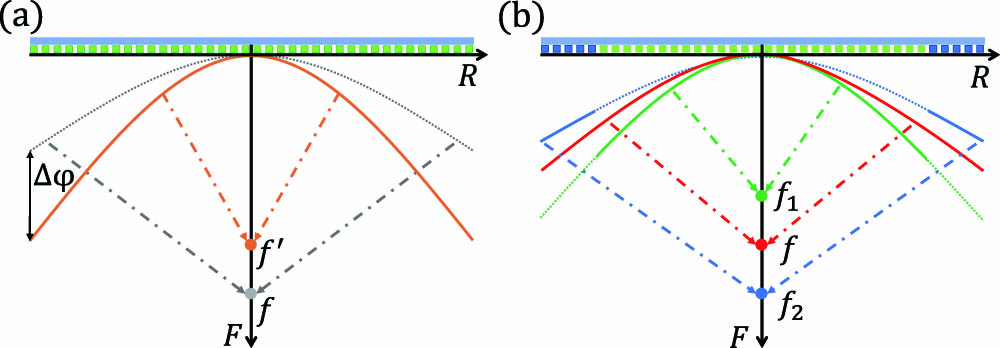
Set citation alerts for the article
Please enter your email address