
- Photonics Research
- Vol. 10, Issue 5, 1308 (2022)
Abstract
1. INTRODUCTION
The generation of optical frequency combs (OFCs) has been of great interest recently as they can provide equidistant mutually locked coherent modes, which make them ideal sources for precision metrology, data communications, and optical interconnects. Over the past decades, various approaches have been extensively investigated to realize on-chip OFCs, such as IM/phase EO modulation [1–3], soliton microresonators [4–6], gain-switched combs [7,8], and passive mode-locked lasers (MLLs) [9]. Among them, soliton microresonators have drawn significant attention recently due to their compactness and ultra-wide bandwidth. In comparison, semiconductor MLLs have the advantages of being electrically driven, while not requiring external high-power optical pumping, which makes them a great candidate for highly efficient, small-footprint, and low power consumption solutions for optical time-division multiplexing [10] and wavelength-division multiplexing (WDM) systems [11]. In recent years, there has been significant attention paid to quantum dash (Q-dash) [12–14] and quantum dot (QD) MLLs [9,15,16] as flat-top comb sources, due to their properties of small linewidth enhancement factor, fast carrier dynamic-induced self-frequency modulation, and defect insensitivity, which make them an ideal platform for a reflection insensitive [17,18], temperature insensitive [19,20], and most importantly, direct-grown-on-Si light source [21–26].
Conventionally, semiconductor MLLs have been investigated to produce the optical pulses with a wide range of repetition rates from megahertz to hundreds of gigahertz as listed in Table 1. The ultra-dense optical combs (
Comparison of Mode-Locked Comb Laser on Various Material Platforms and Structures
Mode-Locking Mode | Material Platform | Repetition | Optical Bandwidth | Optical | Operating Temp. (°C) | |
---|---|---|---|---|---|---|
[ | Anti-CPM (external cavity) | InGaAsP MQW on Si | 1 | 12 (−10 dB BW) | 400 | 20 |
[ | Hybrid anti-CPM (external cavity) | InGaAsP MQW on Si | 1 | 13 (−10 dB BW) | 400 | 20 |
[ | CPM (external cavity) | InGaAsP MQW on Si | 10 | 6.4 (−3 dB BW) | 16 | |
[ | CPM (external cavity) | MQW on Si | 20 | 2.96 (−3 dB BW) | NA | NA |
[ | Two-section | InAs/GaAs QD on Si | 20 | 6.1 (−3 dB BW) | 10,000 | 18 |
[ | Two-section | InAs/GaAs QD | 25.5 | 4.7 (−6 dB BW) | NA | 20–120 |
[ | Two-section | AlGaInAs/InP MQW | 100 | 8.05 (−3 dB BW) | NA | 20 |
[ | CPM (external cavity) | InAs/GaAs QD on SOI | 102 | 6.5 (−3 dB BW) | NA | 25 |
[ | CPM (external cavity) | InAs/GaAs QD on SOI | 15.5 | 12 (−3 dB BW) | NA | 23 |
In this work, we experimentally demonstrate an O-band 100 GHz InAs/GaAs QD-colliding pulse mode-locked laser (CPML) (fourth order) with a record wide 3 dB optical bandwidth of 11.5 nm and a state-of-the-art working temperature ranging from 20°C to 100°C. By optimizing the operation conditions, we have achieved steady flat-top ML region of 20 comb lines within 3 dB optical bandwidth at 100 GHz mode spacing. The individual comb lines exhibit narrow average optical linewidth of 440 kHz. The measured relative intensity noise of the entire comb spectrum and each channel is
Sign up for Photonics Research TOC. Get the latest issue of Photonics Research delivered right to you!Sign up now
To place our demonstration into context, the performance analyses of mode-locked comb lasers on various platforms have been compiled in Table 1. For external cavity MLLs, QW lasers exhibit relatively narrow optical linewidth, benefitting from their long cavity length [27–29]. But the repetition rates (comb spacings) are also limited within 20 GHz. In contrast, short-cavity two-section MLLs, which have a single saturable absorber at the end of the cavity, would be able to produce comb lines with larger channel spacing, but the optical linewidth is normally within the range of tens of megahertz [30–32]. In addition, high-repetition-rate two-section MLs do also experience low optical gain due to their short cavity property, which leads to a significant degradation in single-channel output power. Here, by incorporating the high-order CPM technique, we could generate a 100 GHz grid comb laser with ultra-wide optical bandwidth (11.5 nm), while retaining 1580 μm cavity length for sufficient output power.
2. DEVICE DESIGN AND FABRICATION
The epitaxy of the QD-CPML is grown by molecular beam epitaxy on an n-type GaAs (100) substrate, containing eight stacks of self-assembled InAs QD layers. The active region of the dots in well structure is p-doped to improve the high-temperature stability of the lasers. A symmetric cladding layer design is used by utilizing 1500 nm thick p-type and n-type
Figure 1.(a) Schematic of InAs/GaAs fourth-order CPML design (total cavity length of 1580 μm) with four gain sections (360 μm for each section) and three equally spaced saturable absorbers (50 μm for each SA). (b) Cross-section SEM image of a fabricated device with a 45° tilted angle. Inset: magnified SEM image on electrical isolation trench region.
The QD-CPMLs are fabricated with ridge waveguide and mesa widths of 4 μm and 25 μm, respectively. The three SAs are equally spaced along the cavity with the length of 50 μm, where the electrical isolation gap next to the SA is designed as 10 μm wide. The QD-CPML is then fabricated in line with the standard ridge waveguide InAs QD lasers by using dry etching, dielectric passivation, and metal contact deposition. The electrical isolation gaps are simultaneously defined with ridge waveguide etching by carefully controlling the dry etching depth at 100 nm above the active region. This process simply avoids additional efforts made to the definition of isolation trenches. The detailed fabrication processes are discussed in our previous work [23–25]. The SEM picture in Fig. 1(b) shows the fabricated QD-CPML device, where the inset shows the zoom-in isolation gap region between the gain section and the SA. The sheet resistance is
3. DEVICE CHARACTERIZATIONS AND DISCUSSION
The fabricated QD-CPML is mounted on an AlN chip-on-carrier (CoC) for heat dissipation, while all the SAs, gain sections, and n contact pads are wire bonded to the CoC with three separated pads. The QD-CPML CoC is then mounted on top of a thermoelectric cooler for constant temperature control. The gain sections are driven by the Keithley 2520 current source and SAs are reverse biased by a voltage source. By using an integration sphere with an InGaAs photodetector to collect optical output power from lasers, the light-current (
Figure 2.(a) Temperature-dependent continuous-wave light current (
The lasers are characterized with gain current (
To investigate an optimum operation window of the fourth-order CPML where stable 100 GHz spacing flat-top OFCs can be generated, a wide range of operation conditions have been systematically mapped with
Figure 3.Fourth-order QD-CPML. (a) Optical spectrum 3 dB bandwidth mapping as a function of SA reverse bias voltage ranging from 0 to 5 V with 0.5 V step and injection current of gain sections varying from 50 to 250 mA with 10 mA step. (b) A precisely swept map of comb line numbers within 3 dB optical bandwidth as a function of SA reverse bias voltage varying from 3 to 4.5 V with 0.1 V step and gain section injection current changing from 210 to 250 mA with 1 mA step [the mapping area corresponds to the red rectangular zone in (a)]. (c) Pulse width and (d) time–bandwidth product (TBP) mapping as a function of SA reverse bias voltage ranging from 0 to 5 V with 1 V step and gain section injection current ranging from 50 to 250 mA with 10 mA step. (e) Optical spectra and (f) pulse AC trace evolutions with injection current from 50 to 250 mA with 10 mA step at
The generation of broadband flat-top combs is the major focus in this paper for high-capacity WDM applications. For the flat-top ML region discussed above, a high-resolution sweep of comb line counts within 3 dB optical bandwidth is performed over an optimum reverse bias range from 3 to 4.5 V with magnified 0.1 V step and varying injection current from 210 to 250 mA under 1 mA step increment. As shown in Fig. 3(b), there is a “riverway”-like unlocked region separating the flat-top comb area; along the left “riverbank,” the comb spectral bandwidth reaches the maximum condition. Two dark red blocks show the best conditions which can generate 20 comb lines within the 3 dB optical bandwidth.
Three operation zones are distinguished here by analyzing the spectral envelope shapes, which are the single-state region, dual-state region, and flat-top region. In addition, the mapping results of pulse width are shown in Fig. 3(c), where the narrowest full width at half-maximum of AC trace (dark red region) is
As injection currents are elevated over 210 mA, the dual-state region was strongly suppressed, while merging back into single-lobe comb spectra as shown in Fig. 3(e). Different from the Gaussian-shape-like spectrum, the optical spectrum is broadened to a rectangular shape with flat profiles, and thus the region is named as flat-top ML. The formation of the flat-top profile is induced from the great compensation of increased Kerr non-linearity at high carrier injection toward increased group velocity dispersion (GVD) across the enlarged spectral bandwidth. Similar mechanisms have been previously discussed theoretically and experimentally in single-section MLLs [44–47]. The results are in line with significantly broadened AC pulse width of a few picoseconds [Fig. 3(c)], indicating an enhanced dispersion process within the flat-top MLL region compared with the single-state MLL region.
To further investigate the ML evolution at fixed reverse bias voltage in both optical spectral and time domains, current dependent optical spectral and normalized pulse width evolutions at
Under the optimum operating condition of
Figure 4.(a) Optical spectrum of flat-top QD-CPML under optimized bias condition of
Figure 4(b) shows the frequency noise spectra of 18 filtered comb lines. By fitting the white noises, the intrinsic Lorentz optical linewidths are estimated and plotted in Fig. 4(a). The average optical linewidth of 18 comb channels is approximately 440 kHz, which is 1 order of magnitude lower than that of the reported conventional QD MLLs [30]. The narrow optical linewidth indicates that the phases of individual comb lines are highly coherent through high-order mode locking. Furthermore, the relative intensity noise (RIN) spectra of each comb line (PDFA amplified) and entire comb spectrum are both characterized. Figure 4(c) shows that the 18 individual channels and whole comb spectrum have average RINs of
High-speed external modulation of the fourth-order CPML is demonstrated by evaluating the eye diagram performance of the laser. The large signal modulation experimental setup for the QD comb laser is shown in Fig. 5(a). The CPML is operated at optimum flat-top conditions as discussed above, then coupled into an OBPF to select individual comb channels as optical carriers. A single channel at 1321.28 nm is selected for testing to show the typical performance. By adjusting the amplification current of the PDFA, the carrier is amplified to 10 dBm and coupled into a 40 GHz lithium niobate (LN) Mach–Zehnder modulator (iXblue MX1300-LN-40) for data modulation. The modulation signals are produced from an arbitrary waveform generator (Keysight M8194A) with a
Figure 5.(a) Experimental setup used to measure B2B NRZ and PAM-4 transmission characteristics of QD-CPML, including ISO, optical isolator; OBPF, optical bandpass filter; PDFA, praseodymium-doped fiber amplifier; PC, polarization controller; AWG, arbitrary waveform generator; RF Amp, RF amplifier; MZM, Mach–Zehnder modulator; OSC, optical sampling oscilloscope. (b) 70 Gbit/s NRZ and (c) 40 GBaud PAM-4 optical eye diagram using comb line at 1321.28 nm.
The total transmission bandwidth can also be enhanced in the aspect of optical bandwidth. Taking advantage of the temperature resilience properties of QD lasers, the optical spectrum could be extended by combining the output optical spectra from the QD laser array where each laser is operated at properly designed temperature. The proof-of-concept experiment has been performed by adjusting the operation temperature of a QD-CPML to seamlessly combine and extend the optical spectra. As shown in Fig. 6, the 6 dB optical bandwidth of OFCs is successfully extended to 36 nm with temperature varying from 15°C to 63°C. The broadened optical bandwidth includes sixty 100 GHz spacing OFCs, which has the potential of increasing the data transmission capacity further to 4.8 Tbit/s.
Figure 6.(a) Combined optical spectra of fourth-order QD-CPML for extended optical bandwidth under the temperatures of 15°C, 25°C, 51°C, and 63°C (purple line:
4. CONCLUSIONS
We have successfully demonstrated an O-band 100 GHz grid fourth-order QD-CPML with a record high 3 dB optical bandwidth of 11.5 nm. Taking advantage of the temperature insensitivity of QDs, the laser could generate flat-top OFCs with average 3 dB bandwidth over 10 nm within a wide temperature range from 20°C to 80°C. Sophisticated analyses of high-order CPMLs are included in this work; both Fourier transfer limited sub-picosecond pulses and flat-top comb can be generated from the QD-CPML at different ML regions. The flat-top comb laser shows excellent integrated average RIN values of the entire spectrum and 18 filtered channels at
Acknowledgment
Acknowledgment. The authors would like to thank Mr. Mingchen Guo, Miss Shujie Pan, and Miss Yiou Cui for their constructive contributions in device fabrications and measurements.
References
[1] R. Wu, V. R. Supradeepa, C. M. Long, D. E. Leaird, A. M. Weiner. Generation of very flat optical frequency combs from continuous-wave lasers using cascaded intensity and phase modulators driven by tailored radio frequency waveforms. Opt. Lett., 35, 3234-3236(2010).
[2] M. Y. Xu, M. B. He, Y. T. Zhu, S. Y. Yu, X. L. Cai. Flat optical frequency comb generator based on integrated lithium niobate modulators. J. Lightwave Technol., 40, 339-345(2021).
[3] S. Liu, K. Wu, L. Zhou, L. Lu, B. Zhang, G. Zhou, J. Chen. Optical frequency comb and Nyquist pulse generation with integrated silicon modulators. IEEE J. Sel. Top. Quantum Electron., 26, 8300208(2019).
[4] P. Delhaye, A. Schliesser, O. Arcizet, T. Wilken, R. Holzwarth, T. J. Kippenberg. Optical frequency comb generation from a monolithic microresonator. Nature, 450, 1214-1217(2007).
[5] T. J. Kippenberg, R. Holzwarth, S. A. Diddams. Microresonator-based optical frequency combs. Science, 332, 555-559(2011).
[6] M. G. Suh, K. J. Vahala. Soliton microcomb range measurement. Science, 359, 884-887(2018).
[7] W. L. Weng, A. Kaszubowska-Anandarajah, J. J. He, P. D. Lakshmijayasimha, E. Lucas, J. Q. Liu, P. M. Anandarajah, T. J. Kippenberg. Gain-switched semiconductor laser driven soliton microcombs. Nat. Commun., 12, 1425(2021).
[8] R. Zhou, S. Latkowski, J. O’Carroll, R. Phelan, L. P. Barry, P. Anandarajah. 40 nm wavelength tunable gain-switched optical comb source. Opt. Express, 19, B415-B420(2011).
[9] E. U. Rafailov, M. A. Cataluna, W. Sibbett. Mode-locked quantum-dot lasers. Nat. Photonics, 1, 395-401(2007).
[10] A. Aboketaf, A. Elshaari, S. Preble. Optical time division multiplexer on silicon chip. Opt. Express, 18, 13529-13535(2010).
[11] D. Kong, H. Xin, K. Kim, Y. Liu, L. K. Oxenloewe, P. Dong, H. Hu. Intra-datacenter interconnects with a serialized silicon optical frequency comb modulator. J. Lightwave Technol., 38, 4677-4682(2020).
[12] Z. Lu, J. Liu, S. Raymond, P. Poole, P. Barrios, D. Poitras. 312-fs pulse generation from a passive C-band InAs/InP quantum dot mode-locked laser. Opt. Express, 16, 10835-10840(2008).
[13] J. Liu, Z. Lu, S. Raymond, P. Poole, P. Barrios, D. Poitras. Dual-wavelength 92.5 GHz self-mode-locked InP-based quantum dot laser. Opt. Lett., 33, 1702-1704(2008).
[14] Z. G. Lu, J. R. Liu, C. Y. Song, J. Weber, Y. Mao, S. D. Chang, H. P. Ding, P. J. Poole, P. J. Barrios, D. Poitras, S. Janz, M. O’Sullivan. High performance InAs/InP quantum dot 34.462-GHz C-band coherent comb laser module. Opt. Express, 26, 2160-2167(2018).
[15] X. Huang, A. Stintz, H. Li, L. F. Lester, J. Cheng, K. J. Malloy. Passive mode-locking in 1.3 μm two-section InAs quantum dot lasers. Appl. Phys. Lett., 78, 2825-2827(2001).
[16] B. Dong, H. Huang, J. Duan, G. Kurczveil, D. Liang, R. Beausoleil, F. Grillot. Frequency comb dynamics of a 1.3 μm hybrid-silicon quantum dot semiconductor laser with optical injection. Opt. Lett., 44, 5755-5758(2019).
[17] J. Duan, H. Huang, B. Dong, J. C. Norman, Z. Zhang, J. E. Bowers, F. Grillot. Dynamic and nonlinear properties of epitaxial quantum dot lasers on silicon for isolator-free integration. Photon. Res., 7, 1222-1228(2019).
[18] J. J. Chen, Z. H. Wang, W. Q. Wei, T. Wang, J. J. Zhang. Sole excited-state InAs quantum dot laser on silicon with strong feedback resistance. Front. Mater., 8, 163(2021).
[19] J. Kwoen, B. Jang, K. Watanabe, Y. Arakawa. High-temperature continuous-wave operation of directly grown InAs/GaAs quantum dot lasers on on-axis Si (001). Opt. Express, 27, 2681-2688(2019).
[20] J. C. Norman, D. Jung, Z. Y. Zhang, Y. T. Wang, S. T. Liu, C. Shang, R. W. Herrick, W. W. Chow, A. C. Gossard, J. E. Bowers. A review of high-performance quantum dot lasers on silicon. IEEE J. Quantum Electron., 55, 2000511(2019).
[21] S. Chen, W. Li, J. Wu, Q. Jiang, M. Tang, S. Shutts, S. N. Elliott, A. Sobiesierski, A. J. Seeds, I. Ross, P. M. Smowton. Electrically pumped continuous-wave III–V quantum dot lasers on silicon. Nat. Photonics, 10, 307-311(2016).
[22] A. Y. Liu, C. Zhang, J. Norman, A. Snyder, D. Lubyshev, J. M. Fastenau, A. W. Liu, A. C. Gossard, J. E. Bowers. High performance continuous wave 1.3 μm quantum dot lasers on silicon. Appl. Phys. Lett., 104, 041104(2014).
[23] Z. H. Wang, W. Q. Wei, Q. Feng, T. Wang, J. J. Zhang. InAs/GaAs quantum dot single-section mode-locked lasers on Si (001) with optical self-injection feedback. Opt. Express, 29, 674-683(2021).
[24] W. Q. Wei, J. Y. Zhang, J. H. Wang, H. Cong, J. J. Guo, Z. H. Wang, H. X. Xu, T. Wang, J. J. Zhang. Phosphorus-free 1.5 μm InAs quantum-dot microdisk lasers on metamorphic InGaAs/SOI platform. Opt. Lett., 45, 2042-2045(2020).
[25] W. Q. Wei, Q. Feng, J. J. Guo, M. C. Guo, J. H. Wang, Z. H. Wang, T. Wang, J. J. Zhang. InAs/GaAs quantum dot narrow ridge lasers epitaxially grown on SOI substrates for silicon photonic integration. Opt. Express, 28, 26555-26563(2020).
[26] Q. Feng, W. Q. Wei, B. Zhang, H. L. Wang, J. H. Wang, H. Cong, T. Wang, J. J. Zhang. O-band and C/L-band III-V quantum dot lasers monolithically grown on Ge and Si substrate. Appl. Sci., 9, 385(2019).
[27] Z. C. Wang, K. V. Gasse, V. Moskalenko, S. Latkowski, E. Bente, B. Kuyken, G. Roelkens. A III-V-on-Si ultra-dense comb laser. Light Sci. Appl., 6, 6260(2017).
[28] V. Corral, R. Guzmán, C. Gordón, X. J. M. Leijtens, G. Carpintero. Optical frequency comb generator based on a monolithically integrated passive mode-locked ring laser with a Mach–Zehnder interferometer. Opt. Lett., 41, 1937-1940(2016).
[29] M. L. Davenport, S. T. Liu, J. E. Bowers. Integrated heterogeneous silicon/III–V mode-locked lasers. Photon. Res., 6, 468-478(2018).
[30] S. T. Liu, X. R. Wu, D. Jung, J. C. Norman, M. J. Kennedy, H. K. Tsang, A. C. Gossard, J. E. Bowers. High-channel-count 20 GHz passively mode-locked quantum dot laser directly grown on Si with 4.1 Tbit/s transmission capacity. Optica, 6, 128-134(2019).
[31] S. J. Pan, J. O. Huang, Z. C. Zhou, Z. X. Liu, L. Ponnampalam, Z. Z. Liu, M. C. Tang, M. C. Lo, Z. Z. Cao, K. Nishi, K. Takemasa, M. Sugawara, R. Penty, I. White, A. Seeds, H. Y. Liu, S. M. Chen. Quantum dot mode-locked frequency comb with ultra-stable 25.5 GHz spacing between 20°C and 120°C. Photon. Res., 8, 1937-1942(2020).
[32] L. P. Hou, Y. G. Huang, Y. H. Liu, R. K. Zhang, J. K. Wang, B. J. Wang, H. L. Zhu, B. Hou, B. C. Qiu, J. H. Marsh. Frequency comb with 100 GHz spacing generated by an asymmetric MQW passively mode-locked laser. Opt. Lett., 45, 2760-2763(2020).
[33] G. Kurczveil, C. Zhang, A. Descos, D. Liang, M. Fiorentino, R. Beausoleil. On-chip hybrid silicon quantum dot comb laser with 14 error-free channels. IEEE International Semiconductor Laser Conference (ISLC), 1-2(2018).
[34] G. Kurczveil, A. Descos, D. Liang, M. Fiorentino, R. Beausoleil. Hybrid silicon quantum dot comb laser with record wide comb width. OSA FiO_LS Virtual Conference, FTu6E.6(2020).
[35] C. Kumar, R. Goyal. Effect of crosstalk in super dense wavelength division multiplexing system using hybrid optical amplifier. J. Opt. Commun., 40, 347-351(2019).
[36] M. Sysak, J. Johnson, D. Lewis. CW-WDM MSA technical specifications Rev 1.0(2021).
[37] Z. Zhang, R. Hogg, X. Lv, Z. Wang. Self-assembled quantum-dot superluminescent light-emitting diodes. Adv. Opt. Photonics, 2, 201-228(2010).
[38] Y. Li, F. Chiragh, Y. Xin, C. Lin, J. Kim, C. Christodoulou, L. Lester. Harmonic mode-locking using the double interval technique in quantum dot lasers. Opt. Express, 18, 14637-14643(2010).
[39] H. Y. Liu, S. L. Liew, T. Badcock, D. J. Mowbray, M. S. Skolnick, S. K. Ray, T. L. Choi, K. M. Groom, B. Stevens, F. Hasbullah, C. Y. Jin. P-doped 1.3 μm InAs/GaAs quantum-dot laser with a low threshold current density and high differential efficiency. Appl. Phys. Lett., 89, 073113(2006).
[40] J. Z. Huang, W. Q. Wei, J. J. Chen, Z. H. Wang, T. Wang, J. J. Zhang. P-doped 1300 nm InAs/GaAs quantum dot lasers directly grown on SOI substrate. Opt. Lett., 46, 5525-5528(2021).
[41] J. K. Mee, R. Raghunathan, D. Murrell, A. Braga, Y. Li, L. F. Lester. Novel optical systems design and optimization XVII. Proc. SPIE, 9193, 919301(2014).
[42] S. G. Li, Q. Y. Gong, Y. F. Lao, H. Yang, S. Gao, P. Chen, Y. G. Zhang, S. L. Feng, H. L. Wang. Two-color quantum dot laser with tunable wavelength gap. Appl. Phys. Lett., 95, 251111(2009).
[43] M. Charis, C. Simos, I. Simos, S. Mikroulis, I. Krestnikov, D. Syvridis. Pulse width narrowing due to dual ground state emission in quantum dot passively mode locked lasers. Appl. Phys. Lett., 96, 211110(2010).
[44] J. Hillbrand, D. Auth, M. Piccardo, N. Opačak, E. Gornik, G. Strasser, F. Capasso, S. Breuer, B. Schwarz. In-phase and anti-phase synchronization in a laser frequency comb. Phys. Rev. Lett., 124, 023901(2020).
[45] N. Opačak, B. Schwarz. Theory of frequency-modulated combs in lasers with spatial hole burning, dispersion, and Kerr nonlinearity. Phys. Rev. Lett., 123, 243902(2019).
[46] S. P. Duill, S. G. Murdoch, R. T. Watts, R. Rosales, A. Ramdane, P. Landais, L. P. Barry. Simple dispersion estimate for single-section quantum-dash and quantum-dot mode-locked laser diodes. Opt. Lett., 41, 5676-5679(2016).
[47] R. Rosales, S. G. Murdoch, R. T. Watts, K. Merghem, A. Martinez, F. Lelarge, A. Accard, L. P. Barry, A. Ramdane. High performance mode locking characteristics of single section quantum dash lasers. Opt. Express, 20, 8649-8657(2012).
[48] N. Eiselt, H. Griesser, M. Eiselt, W. Kaiser, S. Aramideh, J. Olmos, I. Monroy, J. Elbers. Real-time 200 Gb/s (4 × 56.25 Gb/s) PAM-4 transmission over 80 km SSMF using quantum-dot laser and silicon ring-modulator. Optical Fiber Communication Conference, Part F40-O, W4D.3(2017).
[49] M. B. He, M. Y. Xu, Y. X. Ren, J. Jian, Z. L. Ruan, Y. S. Xu, S. Q. Gao, S. H. Sun, X. Q. Wen, L. D. Zhou, L. Liu, C. J. Guo, H. Chen, S. Y. Yu, L. Liu, X. L. Cai. High-performance hybrid silicon and lithium niobate Mach–Zehnder modulators for 100 Gbit s−1 and beyond. Nat. Photonics, 13, 359-364(2019).
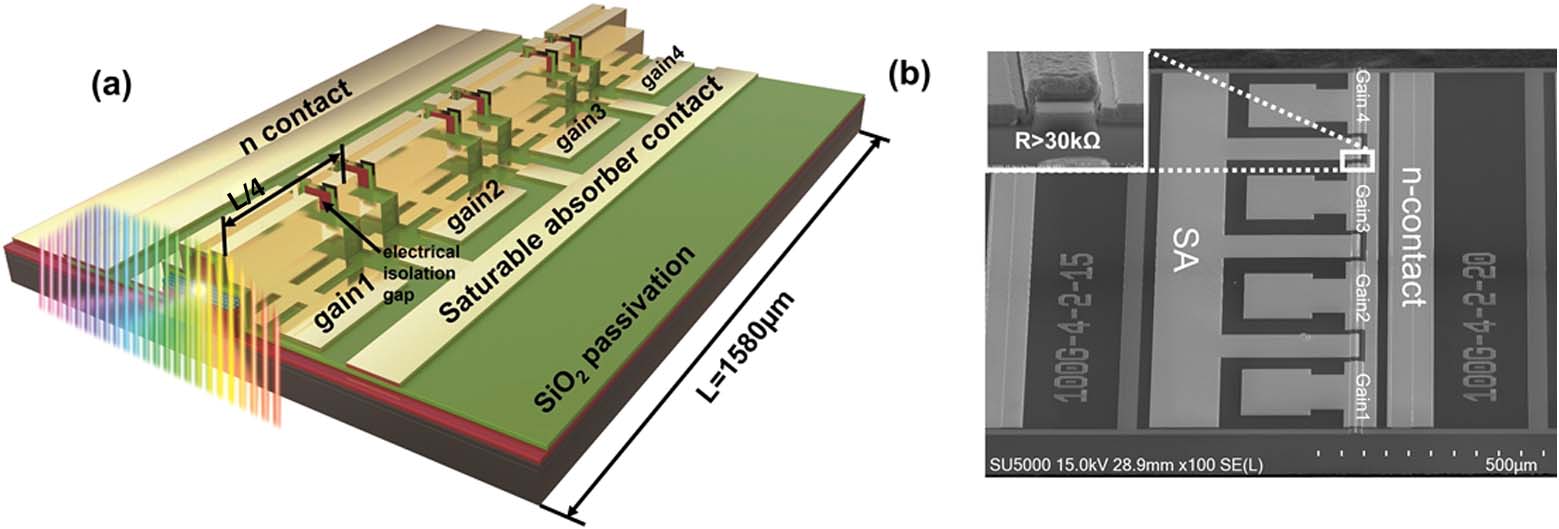
Set citation alerts for the article
Please enter your email address