Author Affiliations
1Nanjing University of Science and Technology, School of Electronic and Optical Engineering, Nanjing, Jiangsu, China2Boston University, Department of Electrical and Computer Engineering, Boston, Massachusetts, United Statesshow less
Fig. 1. Illustration of our aIDT imaging system. (a) A photo of our aIDT system consisting of a standard microscope equipped with an LED ring. A visualization demonstrating the operation of the system is shown in Video 1. (b) An LED ring illumination unit is placed underneath the sample. The distance is tuned such that the illumination angle is matched with the objective NA. (c) Each IDT image measures the interference between the scattered and the unperturbed fields. (d) The absorption and phase TFs at various illumination angles and sample depths (Video 1, MPEG, 2.7 MB [URL: https://doi.org/10.1117/1.AP.1.6.066004.1]).
Fig. 2. Results from our LED position self-calibration method. (a) A sample intensity image of a diatom under a certain single-LED illumination. (b) LED positions from manual alignment (termed uncalibrated, marked in blue star) and our self-calibration method (termed calibrated, marked in green triangle) as plotted in the spatial frequency coordinates. (c)–(e) Comparison of the reconstructed RI slides before and after calibration. (c) , (d) , and (e) . More detailed comparisons across the whole volume are provided in Video 2 (Video 2, MPEG, 2.6 MB [URL: https://doi.org/10.1117/1.AP.1.6.066004.2]).
Fig. 3. RI tomography of S. spiralis. (a)–(c) The maximum RI projection views of the recovered 3-D RI distribution in the , , and planes. (d) Zoom-in on closely packed frustule structures. (e)–(g) Reconstructed 2-D cross sectional RI slices at , 0, and planes. (h), (i) -cross-sectional views of the reconstructed RI. (j) A 3-D rendering view of the reconstructed RI distribution. Additional cross-sectional reconstruction and 3-D rendering view from different perspectives are shown in Video 3. (k)–(n) Line profiles across frustule structures to quantify the reconstructed lateral and axial resolution (Video 3, MPEG, 3.3 MB [URL: https://doi.org/10.1117/1.AP.1.6.066004.3]).
Fig. 4. Single cell RI tomography of unstained human cheek cell clusters. (a) A sample raw intensity image under annular illumination. (b), (c) Reconstructed RI cross-sections demonstrate the sectioning capability enabled by the aIDT. Additional examples are shown in Video 4 (Video 4, MPEG, 2.8 MB [URL: https://doi.org/10.1117/1.AP.1.6.066004.4]).
Fig. 5. Time-lapse in vitro tomographic imaging of C. elegans. (a) Recovered RI slice located at central plane at . The full C. elegans worm reconstruction visualization is shown in Video 5. (b) RI stack section in plane close to the mouth of C. elegans. Buccal cavity of C. elegans is distinguishable (indicated by the white arrows). (c) RI distribution of worm at different planes in the marked red square region at . Time-lapse details are demonstrated in Video 6. (d) Visualizations and RI quantification of the C. elegans internal tissue structures at different time points and axial planes. (e) Depth color coding of 3-D RI measurements of sample in the selected subregion with fixed position in the field of view. Four different time points to illustrate the time lapse results of C. elegans (Video 5, MPEG, 9.7 MB [URL: https://doi.org/10.1117/1.AP.1.6.066004.5]; Video 6, MPEG, 9.5 MB [URL: https://doi.org/10.1117/1.AP.1.6.066004.6]).
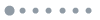
Fig. 6. Simulation for quantifying aIDT accuracy and sensitivity. (a) Top: The object consists of a cuboid array occupying a volume. Bottom: An example aIDT reconstruction. (b) Top: Simulated intensity images with decreasing SNR. Bottom: aIDT reconstructions at . The white box indicates the region over which the cuboid’s RI is obtained for accuracy and sensitivity analysis. (c) Left: aIDT accuracy () evaluations across the object RI. The plot shows the average difference between the aIDT reconstruction and true RI across 100 realizations. Error bars show the standard deviation of this difference. Right: aIDT accuracy across the simulated axial range. aIDT provides accurate RI recovery under low contrast ( to 0.03) objects and loses accuracy from highly scattering features (). The accuracy is stable across the entire reconstruction volume but exhibits fluctuations due to boundary effects in the computation. (d) aIDT-sensitivity () analysis as a function of (left) SNR and (right) axial position under the experimental SNR. aIDT’s sensitivity to small RI changes varies with the object’s RI contrast but maintains to be better than even at high RI contrast for . The sensitivity is constant along for low-contrast objects but fluctuates with increasing RI contrast.