
- High Power Laser Science and Engineering
- Vol. 5, Issue 3, 03000e17 (2017)
Abstract
1 Introduction
Targets are one of the pillars of high-power laser experiments together with the laser facility, diagnostics, and theoretical and numerical tools. In the last decade, target designs have evolved (along with the other pillars) to enable the investigation of new physical phenomena. There are many designs, each specific to the phenomena investigated, the laser parameters and the diagnostic setup. Targets range in size from micrometres to millimetres, not counting possible associated diagnostic shielding, and can range in shape from a homogeneous dot to a layered planar structure, to a 3D object combining multiple shapes and materials. Laser–solid interactions are sensitive to perturbations of the order of the laser wavelength, so these shapes and some of their surfaces must be formed and joined with state-of-the-art precision. Therefore, developing a new target and validating its critical parameters often requires research and development, and different techniques are commonly combined for fabrication of a single-target type. Tens to hundreds of targets are required to support each experimental campaign, since they are usually destroyed in the interaction with the laser pulse.
The demand for such targets will be boosted in the near future by a number of new high-throughput pan-European advanced laser facilities. The High Energy Density (HED) instrument at the European XFEL is expected to start operating for users in 2018 with high-power lasers provided by HIBEF User Consortium (Helmholtz International Beamline for Extreme Fields). The Extreme Light Infrastructure (ELI-Beamlines, ELI Nuclear Physics and ELI-ALPS) is under development and will become operational in the next few years with similar shot rates. The European Synchrotron Radiation Facility (ESRF) has plans for laser-based HED activities, and high repetition rate national laser facilities are or will be soon in operation, e.g., Gemini (United Kingdom), Apollon (France) and CLPU (Spain). All of these facilities promise operation at repetition rates up to 1–10 Hz, corresponding to a requirement of 3600–36,000 targets per hour. Facilities would thus need to provide the supporting technologies for delivering different kinds of targets (such as gas jets, clusters, liquid crystals, and solid targets, some at cryogenic temperature), as well as ensuring the development of manufacturing facilities capable of producing them in the massive numbers and with the needed high precision. Moreover, a number of technological issues will be raised or enhanced by high repetition rate experiments, for example: fast target refreshing, positioning and alignment; real time target characterization and sorting; target debris shielding of laser optics; target cleaning, target chamber nuclear activation, and gas and heat loading of the target chamber. The severity of these issues depends on laser properties, which differ for each class of experiments. For example, the activation and electromagnetic pulses (EMPs) produced by ultra-high-intensity laser pulses for HED experiments are not a problem where intensities below
In this paper, we report on target needs for specific science cases of interest for the high-power laser community (Section
Sign up for High Power Laser Science and Engineering TOC. Get the latest issue of High Power Laser Science and Engineering delivered right to you!Sign up now
2 Target needs
This section gives a general introduction to users’ target needs for three science cases of particular interest for the high-power laser community. Section
2.1 Targets for dynamic compression physics
Dynamic compression physics is one of the largest science fields studied at high-energy laser facilities[
Prototypical experiments for investigating the properties of matter at such extreme pressure and temperature states include equation of state (EOS) measurements, study of high-pressure/high-temperature phase diagrams and new superdense phases[
When investigating matter in extreme states, well-understood optical diagnostics are generally used to determine the conditions that the sample has been driven to. These are primarily Velocity Interferometer System for Any Reflector (VISAR) to measure the shock velocity and transit times and extract the density and pressure from known EOS relations, and Streaked Optical Pyrometery (SOP) for the temperature. More recently, X-ray diagnostics brought new capabilities to further understand the atomic and microscopic structures of the bulk of the compressed matter. On laser only facilities, laser–plasma backlighters can be used to measure X-ray diffraction (XRD), wide angle X-ray scattering (WAXS) and small angle X-ray scattering (SAXS) patterns, as well as absorption spectra in X-ray absorption near edge structure (XANES) and extended X-ray absorption fine structure (EXAFS), or direct imaging by X-rays. With the development of X-ray-free electron lasers (XFELs), energy-resolved scattering has become more easily accessible, using inelastic X-ray scattering (IXS) and X-ray Thomson scattering (XRTS), while still being able to utilize optical diagnostics[
The repetition rate of current shock-compression experiments at combined laser–X-ray facilities is of the order of about 1 shot/10 min to 1 shot/min and the typical number of shots of an experimental campaign is of the order of 100–500. At laser only facilities, the repetition rate can be lower (shot/h or even shot/day), therefore the number of samples needed for an experiment can be considerably lower. The possibility of reaching higher repetition rates (0.1 Hz or better) and collecting data on a larger number of shots would offer new perspectives in this field, for example in the investigation of materials with poor scattering properties (i.e., low-Z materials, liquids), in the study of compression pathways and phase kinetics, and in the collection of data points along the Hugoniot and ramp compression curves. In addition, higher repetition rates would allow for accumulating better statistics for synchrotron radiation and XFEL diagnostics and better spatial as well as spectral resolution for IXS and XRTS, or scanning X-ray parameters such as the X-ray energy for EXAFS.
Target design and optimization are in general carried out by the user group or collaboration. A specific design has to be made to achieve the desired thermodynamic states while taking into account the laser parameters and diagnostics requirements (geometry, atomic and microscopic structures for X-rays, optical windows and properties for VISAR and SOP). Target needs appear quite homogeneous across the community and similar target structures are used by different groups working at both XFEL and synchrotron radiation facilities. Typical targets used for laser compression experiments are either single component foils (whether polycrystalline or single crystal) up to
The advantage of using an ablator, instead of directly ablating the sample, is to confine the laser-produced coronal plasma to the front layer and therefore to reduce gradients in the sample under investigation. Also, a proper choice of the ablation material helps in reaching extreme high pressure as a result of impedance mismatching. Finally it mitigates pre-heating in the sample and helps smoothing of small-scale spatial variations of the laser beam. Typical ablators are plastics, such as Parylene N, polyethylene and polypropylene, or aluminium[
The sample thickness should be optimized for the X-ray diagnostics and laser properties as well as for the time scale of the phenomena under investigation. For example, the attenuation length of X-rays in the target material must be taken into account to avoid loss of signal due to X-ray absorption in the sample. Another important aspect is that the ablator and back window must adhere perfectly to the sample to avoid surface roughness, cracks and porosity and therefore prevent distorted shock front and thermodynamic inhomogeneities. A good adherence is generally obtained with coating techniques or using a thin glue layer. The latter should be avoided at the sample–window interface which is important for VISAR measurements.
Windows are used to act as a tamper and to maintain the thermodynamic conditions avoiding strong release in vacuum. The window should be transparent both to X-rays and visible light for the diagnostics not only at ambient conditions but also under compression (i.e., diamond, which is optimal for X-ray transmission, becomes opaque around 100 GPa). Typical window materials are quartz, lithium fluoride, sapphire and diamond. The window rear face should have an antireflection (AR) coating for the VISAR probe laser.
A high-Z shield layer might be necessary to prevent pre-heating from hard X-rays emitted by the laser-produced coronal plasma.
The thickness of the different layers has to be optimized to ensure that homogeneous thermodynamic conditions are maintained for longer than the time scale of the process to be observed or of the X-ray probe duration (typically several hundreds of ps for synchrotrons and several hundreds of fs for XFELs). In addition, if a sample is too thick, release waves from the ablating material may dramatically reduce the pressure in a portion of the sample. A sandwich target can also be designed to reach off-Hugoniot states or to sustain the peak pressure for longer time (few ns)[
Hydrodynamic codes are currently used to model the wave interactions within the target, such as for example Multi[
Target fabrication and characterization techniques (and the resulting quality, reproducibility and cost of the samples) need to be taken into account in the target design phase. As a consequence, iterations between users and target fabrication groups or companies are needed to develop a final target design. In the optimal case, target production can be completed using coating techniques, as for example: physical vapour deposition (PVD) for metallic films, chemical vapour deposition (CVD) for compounds (including electron beam CVD for oxides and salt structures deposition) and Parylene deposition. However, coating processes only grow layers with thickness up to approximately
2.2 Targets for electron transport and isochoric heating
Investigation of the processes by which energy can be transferred into a dense plasma is, because of the plasma’s opacity, as big a field as the study of the properties of the heated plasma. In this area, energy requirements and time constraints require laser pulses with energy 1–1000 J, pulse length below 1 ps and intensity above
WDM and HED experiments look at the structure and energy flow between the various components of the plasma. Specific HED science questions include understanding the type and growth of instabilities at the plasma surface and energetic particle transport within it, energy interchange between highly energetic particles, ambient electrons, nuclei and photons. These kinds of experiments find application in laboratory astrophysics, study of relativistic plasmas (instabilities), inertial fusion studies, and investigation of the fundamental physics of laser-driven particle and radiation sources (see Section
The number of shots required for electron transport and isochoric heating experiments depends on the specific experiment. In general, tens of shots are needed for tracing the thermal and structural evolution of the system in time. This number can grow if the effect being investigated is small compared to shot-to-shot or sample-to-sample variations, or if the added variance (due to instabilities or chaotic process) is the parameter being studied. Parameter scans are normally performed to determine the dependence of system evolution on initial target properties and laser parameters. For these cases, the total number of shots per campaign might encompass thousands of individual shots. The shot repetition rate in current facilities (typically much lower than
HED and WDM experiments involve targets that are sufficiently large and/or dense for reasonable opacity and that endure for sufficiently long to approach equilibrium. A variety of approaches (singly or in combination) are used for coupling energy in through the surface; for example, cones to concentrate the light and resulting electrons; modulated surface topology, density and/or atomic number to focus EM fields (such modulations can also be produced by fielding with prepulses with known properties). In some cases structures can be built into the target to enable detection of, for example, hot-electron–stimulated fluorescence or a buried layer expansion. X-ray radiation from XFEL or laser-driven (secondary) sources can be used for diagnostics since X-ray scattering techniques are sensitive to nuclear positions and density fluctuations, either thermal or caused by concerted particle motions. Laser-driven proton sources can be used not only to measure electromagnetic fields generated by concerted particle motion, but also to modify the target surface density and atomic number profiles. The use of secondary radiation sources to probe WDM and HED states (pump–probe experiments) requires secondary targets (backlighters) for tailoring or converting the primary (photon) beam to more appropriate excitations (narrow band fluorescence, protons, X-rays). As a result, the completed target assemblies often require addition of a 3D superstructure in the mm scale (multi-target assembly). Target superstructure can also be used for shielding the detector while giving access to the target region under investigation. Figure
Hereinafter, we report a few examples of possible targets exploiting specific geometries or layer sequences for the investigation of isochoric heating and electron transport mechanisms.
Hollow cone structures can be used to guide light and photo-generated electrons into a target at its tip. MeV electrons in the cone are generated via a direct light pressure acceleration mechanism, that increases the number and energy of electrons reaching the cone tip and heating it. For p-polarized radiation (i.e., electric field perpendicular to the cone wall), bunches of electrons are pulled from the cone surface towards the centre of the cone where they are accelerated by the Lorentz force[
WDM states have also been recently studied by irradiating the upper base of cylindrical Ti targets (
Multilayer targets allow not only to study the dependence of electron transport on the material properties, but also to investigate phenomena occurring at the interface between two layers. Electron resistive collimation was investigated using Al targets (transverse size about
Engineered targets can also be used to characterize and understand structural instability growth at a dense plasma surface, that leads to electron filamentation in the bulk of the target (see Figure
Construction of the core of these targets, even micro-cones and complex backlighter targets, is generally compatible with the standard complement of coating and masking techniques used on Si and semiconductors (even though low density foam is sometimes called for and is combined with others only with difficulty). Such an approach has the capability to make many thousands of targets on a single wafer with reasonable cost and increased accuracy[
2.3 Targets for laser-driven particle and radiation sources
The production of laser-driven secondary radiation sources is a very active research area in both long (ns) and short (sub-ps) pulse facilities. A wide range of sources have been investigated in the past decades such as electrons, ions, X-rays (coherent and incoherent), gamma rays and neutrons. A remarkable variety of target types can be used for the generation of secondary radiation, depending on the source type and desired properties, and different challenges must be addressed for the production and characterization of each type of target.
In general, research activities in this field can be classified into two categories. The first (and up to now dominating) category includes exploratory investigation aimed at understanding the basic physics and at improving the source properties, or at generating new types of sources. A large number of experiments in this field are aimed at developing new types of targets to improve the properties or the control of laser-generated radiation and particles. This kind of investigation would benefit from the implementation of high repetition rate laser systems since shot-to-shot variations can be very pronounced. The number of targets required for exploratory studies is a few hundreds (up to 1000) per run. Fast prototyping is essential for this kind of experiment that does not require a huge number of targets of the same type, but rather parametric scans. A second category of experiments is aimed at generating sources with high reproducibility, exploiting the best sources that have been developed in explorative campaigns. The goal of such experiments is to offer particle and radiation sources to users who are not specialists in laser–plasma interactions for applications, for example, in material science, radiobiology and medical science. The generation of secondary particle and radiation sources for applications is one of the goals of some upcoming large-scale facilities such as Apollon, ELI-Beamlines and the LIGHT beamline (Laser Ion Generation, Handling and Transport) at GSI Darmstadt[
Hereinafter, we discuss examples of targets used for the generation of secondary sources and the main challenges for each target type. Established target technologies exist for some types of targets, while improvement (e.g., better modelling, mass production, cost reduction, shaping) or major additional development is required for other target concepts.
2.3.1 Gas targets
Gas targets are mainly used for laser-driven electron acceleration and X-ray production[
Supersonic gas jets (with Mach number up to 10) are the most common type of target for laser-driven electron acceleration experiments. Gas jets have normally high electron density, between
Gas jet targets have also been used to investigate laser-driven ion acceleration occurring in the target volume. Energies of ions produced with low density gas jets are normally in the sub MeV range and show in some cases narrow energy spread[
2.3.2 Solid targets
Solid targets are mainly used for laser-driven ion acceleration and neutron production, even though generation of electron bunches in the interaction of relativistic laser pulses with solid surfaces has been recently observed and attributed to vacuum acceleration of electrons emitted by a plasma mirror[
The most common scheme for laser-driven ion acceleration is known as TNSA and was first observed in 2000 with
Targets for laser-driven ion acceleration range from simple foils (any composition, thickness from tens of nm to tens of
Thin foils have been largely used to investigate TNSA. Parametric scans were performed to investigate the effect of thickness on the acceleration mechanism with different laser contrast ratios[
Since the first observations of TNSA, engineered targets have been used to investigate the acceleration mechanism and characterize the properties of accelerated ions: wedge targets permitted validation of the TNSA model[
surface gratings[
Since TNSA ions are emitted along the rear target normal direction, bent targets have been tested as ion optics: hemispherical, hemicylindrical and hemispherical target coupled with a conic structure[
Multiple target configurations are required for pump–probe laser-driven ion acceleration experiments: laser-driven proton and X-ray radiography were used to diagnose the production of large-scale homogeneous plasmas[
The simplest target configuration for laser-driven neutron generation consists in bulk deuterated polyethylene: DD fusion reactions occur due to collisions between deuterons accelerated at the front target surface with deuterium nuclei in the target bulk (beam fusion, forward directed) or after deuteron thermalization (thermal fusion, isotropic)[
2.3.3 Other target types
Other target types used for laser-driven radiation and particle sources include foams, cryogenic targets, liquid droplets and clusters.
Besides the aforementioned PLD and CVD methods for low density coatings, a number of chemical techniques are commonly used for the production of foam targets, such as sol–gel polymerization, polymerization of the continuous phase of high internal phase emulsions (poly-HIPE) and freeze-dry technique with blowing agents (carbon dioxide or aluminium nitrate). Aerogels and organic acrylic macroporous and mesoporous foams are usually produced by supercritical CO
Liquid crystal targets are produced directly in the interaction chamber by drawing a given volume of liquid crystal (hundreds of nanolitres) with a sharp blade sliding across an aperture on a metal frame[
Cryogenic target devices were initially developed for inertial confinement fusion experiments. This class of targets allows to study laser-driven ion acceleration with pure solid hydrogen or deuterium targets. Several laboratories have been developing systems for in situ formation of cryogenic targets by casting[
Droplet targets have been used for laser-driven ion acceleration as spherical free-standing reduced mass targets (with size of a few
Sub-
3 Target fabrication challenges
The fabrication of solid targets includes many stages, i.e., manufacture of the single components, target assembly from components and mounting on an appropriate carrier device; and target characterization. Figure
As mentioned in Section
Solid targets for high-power laser experiments can be divided into two broad categories: planar and 3D targets. Planar target types include in order of complexity: thin films, multilayers produced by coating techniques, glued multilayers, targets produced on wafer by lithographic techniques (reduced mass targets, modulated surfaces). In general, planar targets can be produced in large sheets by coating techniques (several cm
3D targets are more complex, they can be formed foils or films, as for example hemispheres, or multiple component targets, requiring manual or robotic assembly for each single target. Micromachining techniques are often used for this kind of targets: diamond, laser and electrodischarge machining and drilling; precision lathes and mills. Another potentially interesting technique for target fabrication is 3D printing, that has a typical resolution of
In addition, a number of chemical methods are used for target fabrication, including wet etching, polymer synthesis, production of aerogels, organic and inorganic foams (which require often precision machined mould and critical point drying). Chemical techniques and wet processes allow for reproducible production of large quantities of nanomaterials (with structure size of tens of nm). High-throughput processes for the production of nanostructured targets include, for example, the production of metal oxide dots with block copolymer templates, low density metal foams from alloy or dealloy processes, template-free porous organic films and metal nanoparticles by ultrasonication. Other techniques commonly used are FIB processing, high temperature press, micro-injection moulding, fs laser processing.
In general, creating a target suitable to be shot also requires mounting the targets on holders (often after cutting them) and adding superstructure for alignment and protection to prevent neighbouring targets from damage or modifications due to debris, shock propagation, UV or X-ray emissions, and redeposition of ablated material. Assembly of single components and mounting are often critical steps for multi-target configurations and for 3D targets, and when targets are fragile these operations are preferably done close to the point of use.
Depending on the specific experiment, different final target properties need to be characterized since they can affect the interaction process, the system evolution and the diagnostic efficiency, for example: thickness, density, geometry, composition, crystalline structure, grain size, surface quality (see Figure
Ideally, experimentalists would like to know as much as possible about the properties of each target in order to correlate them with experimental results for each shot. However, most of these techniques are time consuming and this approach is not realistic for large numbers of targets for high repetition rate experiments, unless completely automated characterization processes are developed. In this frame, the most adequate approach is to characterize the production process, rather than each target, and ensure process reproducibility. Therefore, only a few targets from each batch of nominally identical samples has to be characterized to assess the variability of target properties. This information can be used to correlate statistical fluctuations in experimental observations with target property variations and understand what shot-to-shot variation is to be expected due to target variations.
Another critical issue is whether online target characterization is possible and preferable to off-line characterization. The latter is in general more accurate. However, online characterization would allow characterization of the actual properties of the object to be irradiated just before the shot, and to check if any damage has occurred due to the irradiation of neighbouring targets (see Section
Two key issues are related to the demand for large numbers of targets: how to scale production processes to large numbers of targets and how to ensure the reproducibility of target properties. Automated systems for target production, characterization and assembly are probably the only viable solution for both issues. Advantages of automation are high speed assembly over long times, and reproducibility of assembly and handling of delicate parts over long runs that is superior to manual effort (i.e., reduced operator fatigue). Mass production is possible in a few specialized laboratories for micromachining (with integrated confocal microscopy), coating techniques and replication of micromachined surfaces (by using cast replicas as mandrels for plating or by thermal embossing). General Atomics has developed robotic capability for target assembly by using commercial robotic arms augmented with precision X–Y and in some cases Z translation stages used as build platforms. Jigs must be built for each distinct experiment, and assembly steps programmed, therefore considerable initial setup effort is required for each type of assembly. However, incorporation of visual and force feedback to measure the location of randomly placed parts, and development of general purpose jigs and code modules have reduced setup time considerably and the robotic assembling system is now used even for assembly runs of a few hundreds of targets (see Figure
As regards characterization, automation done to date has typically been restricted to single measurements of carefully organized batches. In general, some characterization methods are more amenable to automation than others. For instance, automated dimensional inspection is commercially available in optical coordinate measurement machines (OCMM). Techniques with large standoff distances and atmospheric pressure operating conditions such as optical reflectometry can be automated with pick and place of targets or rastering of targets on palettes. Some systems that require precision adjustment of the instrument to the target have been demonstrated using robotic arms (e.g., automated AFM)[
Robotics expertise is not widely available, nor is its current level of flexibility in setup appreciated in the community. A conscious effort will be needed to provide adequate assembly capability by the time high repetition rate facilities get to full operation (2018–2020). It is also reasonable to expect that operations will emphasize simpler targets and smaller numbers for some time, as operators and experimentalist get used to the opportunities offered by the new capabilities.
4 High repetition rate challenges
As discussed in Section
Currently, high-intensity CPA Ti:sapphire laser systems can run at 10 Hz, with perspective of technological improvement based, for example on fibre technologies that could lead to kHz repetition rates[
In this section, we briefly discuss the main issues related to high repetition rate operation, such as: protection from target debris and neighbouring target damage (Section
4.1 Protection from debris and neighbouring target damage
The production of debris in the laser–matter interaction and the possibility of damage in neighbouring targets are among the most severe problems hindering high repetition rate experiments. High-intensity or high-energy laser pulses normally vaporize several
For planar targets designed as a continuous sheet of material allowing multiple shots, neighbouring target damage can be due to material vaporization (millimetres away from the interaction region), shock waves or even heat waves propagating in the sample for centimetres. Therefore, target holders should be designed to avoid redeposition of evaporated material: the upcoming targets in the target holder must be protected from the vapour and heat generated by shots on the preceding targets and isolated from each other. It is also necessary to prevent the laser beam from directly interacting with the target holder. Laser burn has often been observed on target holders up to 1 cm from the focal spot; the extent of those marks had been put down to imperfect laser focus. The intensity in a perfectly focused laser beam (an Airy disc) decreases as a power law
A possible solution is to separate single shots by a suitable distance, but this is not an option if targets are to be mounted as a single sheet: a 60 cm large sheet of target material would be needed for
In addition, the evaporated material and shrapnel impacting the whole chamber is detrimental to all exposed surfaces, including delicate and expensive components such as optics and diagnostics. Therefore, solutions to mitigate component damage need to be implemented. This issue is relevant also in shot-on-demand experiments, but in this case the vaporization rate is low enough and the off-axis parabola is usually protected with disposable debris shields, to be replaced when damaged or when their transmission is no longer optimal. Currently available debris shields will not be a viable solution for high repetition rate experiments: the degradation rate will be orders of magnitude higher and debris shields will need to be replaced much more often resulting in a waste of time and in increased operation costs, as debris shields can be large and expensive for advanced laser facilities, for example: 550 mm for the 10 PW laser system at ELI-NP, 400 mm for Apollon. The development of cheap and automatic replacement systems for debris collectors and shields is needed. An example is a membrane tape to be rolled progressively as it gets coated, but this solution is not resistant to shrapnel impact: chunks of material accelerated towards the membrane would most likely tear it apart.
Other solutions have been proposed for debris shielding. For example, while plasma is globally neutral, debris could be polarized. Therefore, debris could be collected by polarizing shrapnel and vaporized material and applying an electric field. This technique has been proposed for low energy laser pulses[
In general, the shock, shrapnel, and debris can be minimized by shrinking the actual target to an area that will be surely vaporized and supporting it with ultrathin films, thin micromachined structures or high stiffness fibres. This would also ease chamber cleaning procedures and any health hazards associated to nano-dispersions. Figure
4.2 Target positioning and alignment
In general, target handling devices should be designed to take into account issues, such as: accessibility for diagnostics, first neighbour damage, redeposition of evaporated material, target and holder damage from unfocused laser radiation, X-rays, EMP effects and holder activation. In addition, a crucial requirement for high repetition rate experiments is that the sample must be positioned with
Hereinafter, a few possible concepts for target positioning systems are illustrated. Several options are being considered, including: planar target holders, tape targets, and targets carried on strips and belts.
Planar target delivery systems allow positioning of targets by rastering or rotating a holder containing arrays of samples. This geometry is optimal when the targets are produced as a continuous sheet of material, such as a thin foil or a wafer. Fiducial marks are often used to measure the exact position of the holder and adjust it with micrometre precision. This type of delivery system often allows the mounting of a target holder (specifically designed for a type of target) in a standard frame usually connected to stepper motors.
The High Repetition Rate Sample Delivery (HIREP) working package of the European Cluster of Advanced Laser Light Sources (EUCALL) is developing an integrated concept for decentralized sample characterization and fast sample replacement. The system is composed of a flat carrier frame (specific to each partner facility) and an inner target frame common for all partner facilities (including the ELI pillars, European XFEL, HZDR, Max IV, Desy)[
In general, a possible concern with the flat carrier target scheme, with open area of 10–20 cm is that the density of targets in the carrier is limited to hundreds for full energy shots. At 10 Hz, each carrier is exhausted in about a few minutes.
The High Accuracy Microtarget Supply (HAMS) is a 7 axis target supply system that is being developed by the Central Laser Facility and Scitech Precision Ltd for use on beamlines[
A completely different geometry is based on 1D arrays of targets (scaled up target ladders) for auto-exchanging and limiting the intrusion of the protective shroud. One can store about 1000 ladders, each containing about 200 targets in a cylindrical cassette 40 cm diameter by 100 cm long. Rotation of the cassette puts a selected ladder on a frame that is pulled out by a motorized arm. Only the un-shot targets must be shielded, allowing better diagnostic access than possible with planar arrays. Short-range piezo-motors can position the ladder at laser focus. There is a short interruption in the shots after a ladder is completed when the arm drops the empty ladder and reaches to fetch a new one. Figure
General Atomics is developing a high repetition target fielding scheme based on mechanisms in a cinema film projector (shown in Figure
Finally, thin foils which are robust enough to be wound onto a reel can be positioned by tape drive target delivery systems. The working principle of tape drive systems is quite simple: the target is positioned in the laser focus by spooling the tape. Repetition rates up to 1 kHz have been demonstrated[
4.3 EMPs
The generation of EMPs is a consequence of the interaction of high-power laser pulses with solid matter and can produce severe problems for electronic devices located in the experimental room. The EMP impinging on electronic devices generates oscillating currents in the electronic components and can damage the internal circuits of electronic devices. The coupling of EMP radiation with electronic devices in the experimental room interferes with the correct operation of the devices, producing additional noise in detector readouts and disturbing motor operation. The electronic systems themselves can become an important source of EMP[
EMP are generated as a consequence of the propagation of laser-generated relativistic electrons and return currents that balance the resulting space charge distribution[
Experimental investigation of EMP requires a collection of current probes to measure currents flowing through the target and of B-Probes and D-Probes to measure magnetic and electric fields in different positions in the vacuum chamber (i.e., at different distances from the target and under different angles)[
Precautions to mitigate collateral effects of EMP include, for example: using shielded cables; shielding electronic devices, even though a complete shielding would be required to insulate a component from the interaction region (not feasible for motors and diagnostics); avoiding the formation of loops in cables and electronic devices, which could act as efficient receiving antennas; using insulating feedthroughs, which is only useful when the unwanted current flow along the cable and electronic devices in the target chamber is lower than the predicted current coming from the vacuum chamber wall; appropriate choice of device positions inside and outside the target chamber in order to minimize exposition to direct radiation[
4.4 Other issues
Secondary radiation produced in the laser–matter interaction can induce nuclear reactions in the interaction chamber and beam dumps. Material activation in the interaction chamber equipment and beam dumps could become a critical issue for high repetition rate facilities. For example, in the ELI-NP case, calculations show that the activation produced by a source term of protons with 500 MeV average energy, driven by the 10 PW laser beam and operated 300 min/day (with repetition rate of 1/60 Hz) for 15 consecutive operation days, is such that 5 h are required for the dose rate to decay to
Backreflection of laser light in the laser chain could become a serious issue with high repetition rate operation and progressively improved laser performances. In the interaction of high-intensity laser pulses with solid targets, the plasma produced by the incoming pulse can be pushed inwards by the radiation pressure acting as a piston and boring a hole with transverse dimensions comparable to the incident light spot size[
5 Current target supply strategies and future perspectives
Target supply is a crucial step in the design and implementation of a high-power laser experiment and requires substantial and broad experience in plasma physics and target fabrication. Therefore, it is imperative that experimentalists develop designs in concert with experienced fabricators, and the evaluation of proposals take into account the effort and time needed for target delivery, adjusting upcoming shot schedules as needed. Access to target fabrication expertise is often limited by manpower availability and high costs (up to several
In this respect, the USA Department of Energy (DOE) provides an extensive target supply infrastructure for American facilities and users. DOE supports target supply by funding national laboratories, contractors and universities. A significant fraction of the USA target fabrication is carried out by the Inertial Fusion Technologies (IFT) division of General Atomics, which also designs and builds target fielding equipment. General Atomics IFT employs 110 scientists and technicians, about 40% of whom are stationed at the laser facilities NIF, Z, Omega, to provide support to users in target design and assembly. General Atomics is funded by National Nuclear Security Administration (Contract DE-FC02-04ER54698), to enable laboratories and academic users of DOE facilities access to greater depth of target fabrication capabilities. Master task agreements between General Atomics and national laboratories facilitate additional support from General Atomics. Therefore, industrial target support has a central role in the DOE target supply strategy. In this scheme, the responsibility for target production and R&D activities is shared between academic groups, national laboratories and contactors, with academic groups and national laboratories leaning more towards target fabrication technique development, while General Atomics’ activities are more focused on target production. An ongoing technology transfer between these parties allows increased efficiency, reduced effort duplication and increased overall success of DOE facilities, by making available to all laboratories solutions developed by each partner. The allocation of General Atomics efforts for target fabrication is negotiated with DOE and planned according to the anticipated experiments and the coming year’s shot plan for programme targets and laboratory basic science targets. In addition, a fixed portion of the GA target contract is allocated to target production for the National Laser User Facility (NLUF) programme by which DOE provides access and funding to outside users (e.g., universities) to the OMEGA facility. As a consequence, General Atomics is involved in the proposal evaluation process, providing feedback on target cost and feasibility. Target description, fabrication processes and experiment information stored in a centralized record of concurrence tracking also target request and specification changes (which need to be approved by General Atomics, the laboratory coordinator and the experiment principal investigator). Therefore a database of targets produced for previous experiments is available.
The European community, on the contrary, has no coordinated strategy to access target fabrication infrastructure, and the initiative is left to the individual facilities, institutes and research groups. Some facilities, as for example the Central Laser Facility at Rutherford Appleton Laboratory (RAL) in the United Kingdom, and the PHELIX facility at GSI, Germany make their target fabrication capabilities available to the community through commercial contracts or scientific collaborations. In particular, RAL offers support to CLF users for target supply (within a given budget) thanks to an established target fabrication group. This group is involved in the whole process, from proposal evaluation and technical feasibility assessment to target delivery. The CLF resources are complimented by the capabilities of Scitech Precision Ltd a spin out from the CLF Target Fabrication group. This company has key expertise in certain target manufacture processes (e.g., techniques for the production of micro-electro-mechanical systems) that are made available to the CLF. The company is also a vehicle for the wider community to access the capabilities of both Scitech and the CLF. The CLF and Scitech operate in custom designed cleanroom facilities at the Rutherford Appleton Laboratory and have many years’ experience in delivering user experiments. Scitech collaborates in a wider field with facilities such as the Diamond Light Source in the United Kingdom and is developing techniques and delivery processes that will be transferrable to laser beamlines. Extensive capabilities are present also in France, where the Commissariat à l’Énergie Atomique (CEA) branch in Valduc provides programme targets for ICF, shock-ignition and shock-compression physics. Other facilities, as for example the Laboratoire d’Utilization des Laser Intenses, LULI, provide support in target assembly but rely mainly on academic collaborations for target supply. As regards the upcoming pan-European facilities, European XFEL is planning to have a target preparation area with limited capabilities for target processing and characterization. The European XFEL provides about 300
In addition to facilities and established target fabrication groups, a large number of techniques and extensive competencies for target production and characterization are available in the community. In the last few years a few academic groups focused their research interests on engineered targets, working on target design and on the development of appropriate characterization and fabrication techniques, and testing specific target designs in experiments. Some of these groups have been recently awarded European Research Council grants, for example, to the projects
In the Russian landscape, the Lebedev Physics Institute (LPI) of the Russian Academy of Science in Moscow offers extensive capabilities and experience coming from the thermonuclear research area, ranging from the development of advanced cryogenic targets to low density material production and characterization. Low density targets developed at LPI include polymeric foams (also with density gradients or inclusion of nanoparticles), metallic foams and nanosnow layers. Considerable effort has been devoted to the development of cryogenic systems for high-throughput fabrication of fuel capsules for ICF, including: filling and layering modules for fuel capsules, fast assembly systems for target and protective sabot, target injectors and a system for online characterization of flying targets. Target development is carried out in the frame of several well-established collaborations with other institutes of the Russian Academy of Science, including the A. A. Dorodnitsyn Computer Center, the Institute of Design Problems in Microelectronics, the Institute for High Temperatures and the A. N. Nesmeyanov Institute of Organoelement Compounds. Research activities are also performed in collaboration with local universities (Moscow Engineering Physics Institute – National Research Nuclear University and M. V. Lomonosov Moscow State University), national research institutes, for example, the National Research Center ‘Kurchatov Institute’, and companies such as Inter RAO UES and Cryotrade Ltd.
One of the most important Chinese suppliers is the Target Science and Fabrication Group of the Chinese Academy of Engineering Physics, based in Mianyang, Sichuan. The group focuses mainly on the production of targets for fusion and HED and provides almost all targets for ICF studies and Z-pinch in China. Other institutes, such as Peking University, Beijing, and Shanghai Institute of Optics and Fine Mechanics (SIOM), have recently started developing some in-house capability. In Japan, most target development activities are performed by university groups. The Institute of Laser Engineering (ILE) at Osaka University is the largest and oldest high-power laser facility equipped with a target fabrication group, which provides almost all types of targets for laser experiments. The Kansai Photon Science Institute (National Institutes for Quantum and Radiological Science and Technology) focuses on high repetition rate experiments with the development of gas puff targets and tape targets. Gifu University and Hiroshima University focus on coil injection and gas acceleration, respectively[
As mentioned before, the upcoming European facilities lack a common infrastructure. Therefore, the formulation of a common strategy and the identification of possible synergies would be a key asset to develop a sustainable target supply chain in Europe. The following partners should be involved: established laboratories specialized in target production, material science centres, currently operating and upcoming facilities, university research groups with specific competence, and industry. Potentially, the development of a sustainable supply mechanism could be achieved by better coordinating and increasing the capabilities already available in the community. In addition, the target fabrication community could be enlarged by involving material science laboratories and groups not directly working on laser–plasma activities.
Different synergy levels could be considered depending on the commitment of the community. The lowest synergy level would be based on ‘know-how’ sharing and bilateral collaborations between target laboratory institutes and experimental groups for specific experiments. This synergy is already exploited quite efficiently and exchanges between different groups are promoted by several workshop series. In the European landscape, a consortium of European target suppliers and specialized laboratories (Target Suppliers Network) has recently been formed to coordinate dissemination activities and know-how sharing at the European level[
6 Conclusions
Targets are a key element of every high-power laser experiment and their design is an art that melds broad knowledge of materials properties, materials engineering, and plasma physics. Early involvement of fabricators – ideally before a proposal is submitted – is crucial to ensuring that targets can be fabricated within necessary specifications, are safe for the facility, and will deliver appropriate physics measurements. Target fabrication is a complex process and often requires the combination of different techniques, from material science and chemistry to metrology and engineering. As with diagnostics and optics, target supply requires planning, development and a dedicated budget, especially for high repetition rate facilities requiring huge numbers of targets. Scaling target production, characterization and assembly to large numbers of targets (as required for high repetition rate experiments) will require a high degree of automation. Although some groups are developing automated target processing and applying robotics to target fabrication, these capabilities are not widespread in the community. In general, surprising target fabrication capabilities and competence are available in university groups, material science laboratories and companies traditionally not considered as target suppliers. A better coordination and enhancement of those capabilities, together with the competence of experienced target fabricators, would help in creating a sustainable target supply mechanism for the upcoming facilities. In addition, user facilities should develop very basic production capabilities or, at least, adequate assembly stations, and a target database to keep track of processes and issues. Training of the next generation of target experts should be a priority for facilities and suppliers to ensure the availability of qualified experts and the continuity of laboratory operation.
Another bottleneck for the full exploitation of the potential of upcoming advanced laser facilities could originate from technical issues limiting high repetition rate fielding of solid targets. The availability of technological solutions to enable high repetition rate irradiation of solid targets is a necessary condition for the success of most of the upcoming facilities. Issues related to high repetition rates could be addressed in joint research activities and collaborations between facilities, users and target experts. These activities would require facility access to study the physical phenomena producing the issues and to test possible solutions.
References
[1] B. A. Remington, R. E. Rudd, J. S. Wark. Phys. Plasmas, 22(2015).
[2] Y. B. Zeĺdovich, Y. P. Raizer. Physics of Shock Waves and High-Temperature Hydrodynamic Phenomena(1966).
[6] D. Kraus, J. Vorberger, D. O. Gericke, V. Bagnoud, A. Blažević, W. Cayzac, A. Frank, G. Gregori, A. Ortner, A. Otten, F. Roth, G. Schaumann, D. Schumacher, K. Siegenthaler, F. Wagner, K. Wünsch, M. Roth. Phys. Rev. Lett., 111(2013).
[7] V. E. Fortov. Extreme States of Matter on Earth and in the Cosmos(2011).
[8] T. Guillot. Science, 286, 5437(1999).
[10] D. Kraus, A. Ravasio, M. Gauthier, D. O. Gericke, J. Vorberger, S. Frydrych, J. Helfrich, L. B. Fletcher, G. Schaumann, B. Nagler, B. Barbrel, B. Bachmann, E. J. Gamboa, S. Göde, E. Granados, G. Gregori, H. J. Lee, P. Neumayer, W. Schumaker, T. Döppner, R. W. Falcone, S. H. Glenzer, M. Roth. Nat. Commun., 7(2016).
[12] M. D. Knudson, M. P. Desjarlais. Phys. Rev. Lett., 103(2009).
[13] R. F. Smith, J. H. Eggert, R. Jeanloz, T. S. Duffy, D. G. Braun, J. R. Patterson, R. E. Rudd, J. Biener, A. E. Lazicki, A. V. Hamza, J. Wang, T. Braun, L. Benedict, P. M. Celliers, G. W. Collins. Nature, 511, 7509(2014).
[15] W. J. Nellis, S. T. Weir, A. C. Mitchell. Phys. Rev. B, 59, 3434(1999).
[18] L. B. Fletcher, H. J. Lee, T. Döppner, E. Galtier, B. Nagler, P. Heimann, C. Fortmann, S. LePape, T. Ma, M. Millot, A. Pak, D. Turnbull, D. A. Chapman, D. O. Gericke, J. Vorberger, T. White, G. Gregori, M. Wei, B. Barbrel, R. W. Falcone, C.-C. Kao, H. Nuhn, J. Welch, U. Zastrau, P. Neumayer, J. B. Hastings, S. H. Glenzer. Nat. Photon., 9, 274(2015).
[20] D. Swift, R. Krauss. Phys. Rev. E, 77(2008).
[22] R. Ramis, R. Schmalz, J. Meyer-ter-vehn. Comput. Phys. Commun., 49, 475(1988).
[23] J. P. Colombier, P. Combis, F. Bonneau, R. Le Harzic, E. Audouard. Phys. Rev. B, 71(2005).
[24] J. T. Larsen, S. M. Lane. J. Quant. Spectrosc. Radiat. Transfer, 51, 179(1994).
[25] R. Ramis, J. Meyer-ter-Vehn, J. Ramírez. Comput. Phys. Commun., 180, 977(2009).
[27] T. Kluge, T. Cowan, A. Debus, U. Schramm, K. Zeil, M. Bussmann. Phys. Rev. Lett., 107(2011).
[28] B. S. Paradkar.
[29] M. E. Glinsky. Phys. Plasmas, 2, 2796(1995).
[30] A. R. Bell, J. R. Davies, S. Guerin, H. Ruhl. Plasma Phys. Control. Fusion, 39, 653(1997).
[31] L. G. Huang, T. Kluge, T. E. Cowan. Phys. Plasmas, 23(2016).
[36] A. Schöenlein, G. Boutoux, S. Pikuz, L. Antonelli, D. Batani, A. Debayle, A. Franz, L. Giuffrida, J. J. Honrubia, J. Jacoby, D. Khaghani, P. Neumayer, O. N. Rosmej, T. Sakaki, J. J. Santos, A. Sauteray. EPL, 114(2016).
[38] L. Huang, M. Bussmann, T. Kluge, A. L. Lei, W. Yu, T. E. Cowan. Phys. Plasmas, 20(2013).
[40] T. Kluge, J. Metzkes, K. Zeil, M. Bussmann, U. Schramm, T. E. Cowan. Phys. Plasmas, 22(2015).
[41] C. Spindloe, G. Arthur, F. Hall, S. Tomlinson, R. Potter, S. Kar, J. Green, A. Higginbotham, N. Booth, M. K. Tolley. J. Phys.: Conf. Ser., 713(2016).
[42] G. Arthur. J. Phys.: Conf. Ser., 713(2016).
[43] C. Spindloe, M. K. Tolley, P. Hiscock, M. Beardsley, J. J. Spencer. Fus. Sci. Technol., 59, 221(2011).
[46] P. Gibbon. Short pulse laser interactions with matter(2004).
[47] S. M. Hooker. Nat. Photon., 7, 775(2013).
[49] X. Wang, R. Zgadzaj, N. Fazel, Z. Li, S. A. Yi, X. Zhang, W. Henderson, Y. Y. Chang, R. Korzekwa, H. E. Tsai, C. H. Pai, H. Quevedo, G. Dyer, E. Gaul, M. Martinez, A. C. Bernstein, T. Borger, M. Spinks, M. Donovan, V. Khudik, G. Shvets, T. Ditmire, M. C. Downer. Nat. Commun., 4, 1988(2013).
[55] F. Sylla, M. Veltcheva, S. Kahaly, A. Flacco, V. Malka. Rev. of Sci. Instrum., 83(2012).
[62] A. Macchi, M. Borghesi, M. Passoni. Rev. Mod. Phys., 85, 751(2013).
[66] S. V. Bulanov, E. Y. Echkina, T. Z. Esirkepov, I. N. Inovenkov, M. Kando, F. Pegoraro, G. Korn. Phys. Rev. Lett., 104(2010).
[68] A. Henig, S. Steinke, M. Schnürer, T. Sokollik, R. Hörlein, D. Kiefer, D. Jung, J. Schreiber, B. M. Hegelich, X. Q. Yan, J. Meyer-ter-Vehn, T. Tajima, P. V. Nickles, W. Sandner, D. Habs. Phys. Rev. Lett., 103(2009).
[69] I. J. Kim, K. H. Pae, C. M. Kim, H. T. Kim, J. H. Sung, S. K. Lee, T. J. Yu, I. W. Choi, C.-L. Lee, K. H. Nam, P. V. Nickles, T. M. Jeong, J. Lee. Phys. Rev. Lett., 111(2013).
[75] T. Z. Esirkepov, S. V. Bulanov, K. Nishihara, T. Tajima, F. Pegoraro, V. S. Khoroshkov, K. Mima, H. Daido, Y. Kato, Y. Kitagawa, K. Nagai, S. Sakabe. Phys. Rev. Lett., 89(2002).
[82] M. Passoni, A. Sgattoni, I. Prencipe, L. Fedeli, D. Dellasega, L. Cialfi, I. W. Choi, I. J. Kim, K. A. Janulewicz, H. W. Lee, J. H. Sung, S. K. Lee, C. H. Nam. Phys. Rev. AB, 19(2016).
[84]
[86] A. Zani, D. Dellasega, V. Russo, M. Passoni. Carbon, 56, 358(2013).
[87] I. Prencipe, D. Dellasega, A. Zani, D. Rizzo, M. Passoni. Sci. Technol. Adv. Mater., 16(2015).
[95] S. N. Chen, T. Iwawaki, K. Morita, P. Antici, S. D. Baton, F. Filippi, H. Habara, M. Nakatsutsumi, P. Nicolaï, W. Nazarov, C. Rousseaux, M. Starodubstev, K. A. Tanaka, J. Fuchs. Sci. Rep., 6(2016).
[98] S. Karsch.
[100] D. Klir, P. Kubes, M. Paduch, T. Pisarczyk, T. Chodukowski, M. Scholz, Z. Kalinowska, B. Bienkowska, L. Karpinski, J. Kortanek, J. Kravarik, K. Rezac, I. Ivanova-Stanik, K. Tomaszewski, E. Zielinska. Plasma Phys. Control. Fusion, 54(2011).
[103] W. Nazarov, P. G. McGivern, M. Theobald. Fusion Technol., 38, 110(2000).
[104] J. Andre, R. Botrel, J. Schunck, A. Pinay, C. Chicanne. Fusion Sci. Technol., 70, 237(2016).
[108] F. Ito, N. Nakamura, T. Norimatsu, K. Nagai. Plasma Fusion Res., 4, S1011(2009).
[110] K. Nagai, T. Norimatsu, Y. Izawa. Fusion Sci. Technol., 45, 79(2004).
[111] A. Nikroo, D. Czechowicz, R. Paguio, A. L. Greenwood, M. Takagi. Fusion Sci. Technol., 45, 84(2004).
[112] R. W. Pekala. J. Mater. Sci., 24, 3221(1989).
[113] W. L. Perry, R. C. Dye, P. G. Apen, L. Foreman, E. Peterson. Appl. Phys. Lett., 66, 314(1995).
[114] J. F. Hund, R. R. Paguio, C. A. Frederick, A. Nikroo, M. Thi. Fusion Sci. Technol., 49, 669(2006).
[117] S. Garcia, D. Chatain, J. P. Perin. Laser Part. Beams, 32(2014).
[118] S. Astbury, S. Bedacht, P. Brummitt, D. Carroll, R. Clarke, S. Crisp, C. Hernandez–Gomez, P. Holligan, S. Hook, J. S. Merchan, D. Neely, A. Ortner, D. Rathbone, P. Rice, G. Schaumann, G. Scott, C. Spindloe, S. Spurdle, A. Tebartz, S. Tomlinson, F. Wagner, M. Borghesi, M. Roth, M. K. Tolley. J. Phys.: Conf. Ser., 713(2016).
[127] A. Täschner, E. Köhler, H.-W. Ortjohann, A. Khoukaz. Nucl. Instrum. Methods A, 660, 22(2011).
[131] Z. Gan, Y. Cao, R. Gan, R. Z. Evans, M. Gu. Nat. Commun., 4, 2061(2013).
[132] L. C. Carlson, H. Huang, N. Alexander, J. Bousquet, M. Farrell, A. Nikroo. Fusion Sci. Technol., 70, 274(2016).
[133] G. Mourou, B. Brocklesby, T. Tajima, J. Limpert. Nat. Photon., 7, 258(2013).
[134] E. Hecht, A. Zajac. Optics(1974).
[135] C. K. Park, D. F. Farson. J. Appl. Phys., 118(2015).
[136] K. Fahy, F. O’Reilly, E. Scally, P. Sheridan.
[138] R. W. Moir.
[140] N. Booth, R. Clarke, R. Heathcote, D. Neely, R. Pattathil, D. Rusby, C. Spindloe, D. Symes, M. Tolley, S. Tomlinson. Proc. SPIE, 9211(2014).
[141] D. R. Symes, N. Booth, M. Baraclough, G. Indorf, P. Oliver, G. G. Scott, D. Neely, C. Spindloe, R. I. Heathcote, R. J. Clarke, P. S. Foster, C. D. Gregory, P. P. Rajeev.
[142] N. B. Alexander, D. T. Goodin, R. B. Stephens. Fusion Sci. Technol., 51, 564(2007).
[144] S. J. Haney, K. W. Berger, G. D. Kubiak, P. D. Rockett, J. Hunter. Appl. Opt., 32, 6934(1993).
[145] C. G. Brown, E. Bond, T. Clancy, S. Dangi, D. C. Eder, W. Ferguson, J. Kimbrough, A. Throop. J. Phys.: Conf. Ser., 244(2010).
[147] S. Barbarino, F. Consoli. IEEE Trans. Antennas Propag., 58, 4074(2010).
[149] P. Mora. Phys. Rev. Lett., 90(2003).
[150] R. F. Benjamin, G. H. McCall, A. W. Ehler. Phys. Rev. Lett., 42, 890(1979).
[153] F. S. Felber. Appl. Phys. Lett., 86(2005).
[154] J. Krása, J. Cikhardt, M. De Marco, D. Klír, A. Velyhan, K. Rezac, M. Pfeifer, E. Krousky, J. Skala, R. Dudzak, J. Dostal, J. Kaufman, J. Ullschmied, J. Limpouch.
[155] M. De Marco, M. Pfeifer, E. Krousky, J. Krasa, J. Cikhardt, D. Klír, V. Nassisi. J. Phys.: Conf. Ser., 508(2014).
[156] M. De Marco, J. Cikhardt, J. Krása, A. Velyhan, M. Pfeifer, E. Krouský, D. Klír, K. Řezáč, J. Limpouch, D. Margarone, J. Ullschmied. Nukleonika, 10, 1515(2015).
[157] A. Poyé, S. Hulin, M. Bailly-Grandvaux, J. L. Dubois, J. Ribolzi, D. Raffestin, M. Bardon, F. Lubrano-Lavaderci, E. d’Humiéres, J. J. Santos, P. Nicolaï, V. Tikhonchuk. Phys. Rev. E, 91(2015).
[158] A. Poyé, J.-L. Dubois, F. Lubrano-Lavaderci, D. Raffestin, J. Ribolzi, J. Gazave, A. C. La Fontaine, E. d’Humières, S. Hulin, Ph. Nicolaï, V. T. Tikhonchuk41st EPS Conference on Plasma Phys.
[159] A. Poyé, J.-L. Dubois, F. Lubrano-Lavaderci, E. d’Humières, M. Bardon, S. Hulin, M. Bailly-Grandvaux, J. Ribolzi, D. Raffestin, J. J. Santos, Ph. Nicolaï, V. Tikhonchuk. Phys. Rev. E, 92(2015).
[160] J. L. Remo, R. G. Adams, M. C. Jones. Appl. Opt., 46, 6166(2007).
[161] M. De Marco, J. Krása, J. Cikhardt, M. Pfeifer, E. Krouský, D. Margarone, H. Ahmed, M. Borghesi, S. Kar, L. Giuffrida, R. Vrana, A. Velyhan, J. Limpouch, G. Korn, S. Weber, L. Velardi, D. Delle Side, V. Nassisi, J. Ullschmied. J. Instrum., 11(2016).
[163] D. T. Attwood, D. W. Sweeney, J. M. Auerbach, P. H. Y. Lee. Phys. Rev. Lett., 40, 184(1978).
[164] F. Negoita, M. Roth, P. Q. Thirolf, S. Tudisco, F. Hannachi, S. Moustaizis, I. Pomerantz, P. Mckenna, J. Fuchs, K. Sphor, G. Acbas, A. Anzalone, P. Audebert, S. Balascuta, F. Cappuzzello, M. O. Cernaianu, S. Chen, I. Dancus, R. Freeman, H. Geissel, P. Ghenuche, L. Gizzi, F. Gobet, G. Gosselin, M. Gugiu, D. Higginson, E. d’Humi eres, C. Ivan, D. Jaroszynski, S. Kar, L. Lamia, V. Leca, L. Neagu, G. Lanzalone, V. Meot, S. R. Mirfayzi, I. O. Mitu, P. Morel, C. Murphy, C. Petcu, H. Petrascu, C. Petrone, P. Raczka, M. Risca, F. Rotaru, J. J. Santos, D. Schumacher, D. Stutman, M. Tarisien, M. Tataru, B. Tatulea, I. C. E. Turcu, M. Versteegen, D. Ursescu, S. Gales, N. V. Zamfir. Rom. Rep. Phys., 68, S37(2016).
[165] D. Ursescu, G. Cheriaux, P. Audebert, M. Kalashnikov, T. Toncian, M. Cerchez, M. Kaluza, G. Paulus, G. Priebe, R. Dabu, M. O. Cernaianu, M. Dinescu, T. Asavei, I. Dancus, L. Neagu, A. Boianu, C. Hooker, C. Barty, C. Haefner. Rom. Rep. Phys., 68, S11(2016).
[166] C. C. Gheorghiu, V. Leca, D. Popa, M. O. Cernaianu, D. Stutman. J. Instrum., 11(2016).
[167] N. V. Zamfir. Eur. Phys. J. Special Topics, 223, 1221(2014).
[168] T. Norimatsu, D. Harding, R. Stephens, A. Nikroo, R. Petzoldt, H. Yoshida, K. Nagai, Y. Izawa. Fusion Sci. Technol., 49, 483(2006).
[171]
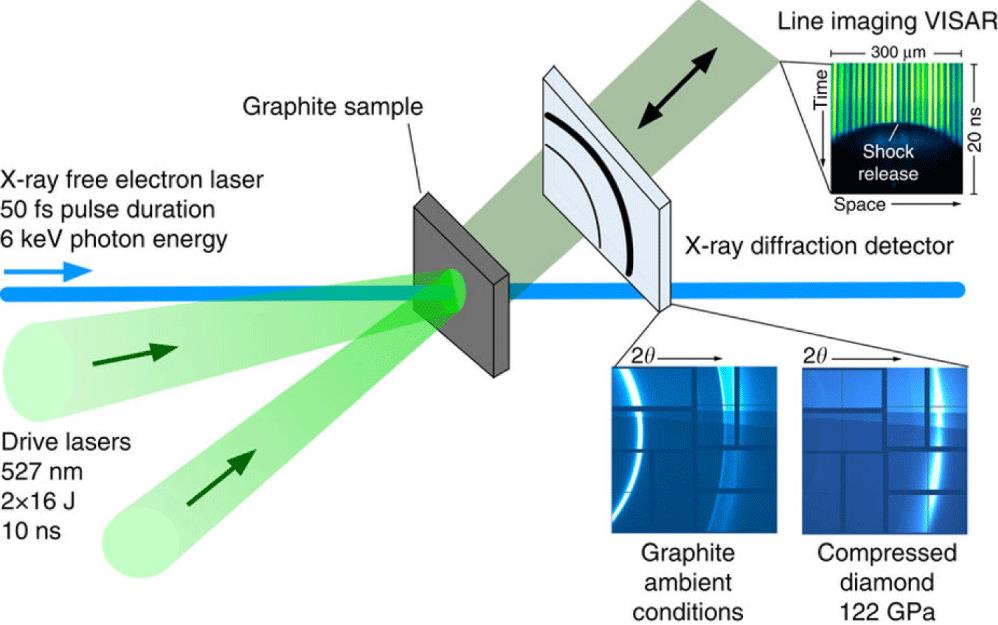
Set citation alerts for the article
Please enter your email address