
- Photonics Research
- Vol. 10, Issue 3, 653 (2022)
Abstract
1. INTRODUCTION
The experimental discovery of topological 3D Dirac semimetals (DSMs) provides unprecedented opportunities for investigating intriguing topological phase transition and various exotic quantum states in condensed matter [1–5]. According to the relative magnitude of potential and kinetic energy components of the Hamiltonian of Dirac fermions, the 3D DSMs are classified to type-I and type-II DSMs [6,7]. The noble transition-metal dichalcogenide (TMD)
In our previous work, the negative terahertz photoconductivity in
Herein, the photoexcited carrier and coherent phonon dynamics in type-II DSM
Sign up for Photonics Research TOC. Get the latest issue of Photonics Research delivered right to you!Sign up now
2. CHARACTERIZATIONS AND EXPERIMENTAL SETUP
Homogeneous and continuous
Ultrafast time-resolved degenerate optical pump-optical probe (OPOP) measurements in transmission configuration were performed with a home-built system. The optical pulses at a central wavelength of 780 nm (1.59 eV) are delivered from a Ti:sapphire regenerative amplifier (Spectra Physics, Spitfire) with single pulse energy of 2 mJ, pulse duration of 120 fs, and repetition rate of 1 kHz. The laser beam was split into the intense pump and weak probe beams, which were focused on the sample, overlapping with the pump spot estimated to be 0.67 mm in diameter, which is roughly twice as large as the probe beam. The fluence of probe light was fixed at about
3. RESULTS AND DISCUSSION
The degenerate 780 nm OPOP measurement was first performed to investigate the excited carrier and coherent phonon dynamics of
Figure 1.Time-resolved transient transmission trace
We now discuss the underlying physical origins that caused the ultrafast optical response. In general, the differential transmission signal
To quantitatively evaluate the quasiparticle relaxation dynamics, a biexponential decay function convoluted with laser pulse width with the below form [24,31] was utilized to fit the transient profile:
Figure 2.Fitting fast lifetime
We now focus on the oscillatory response observed in 20 nm
As a matter of fact, upon ultrafast photoexcitation, the surface layers of material absorb the pump photon, bringing about a transient temperature rise via electron–phonon scattering within the illuminated area. Such sudden temperature elevation sets up localized transient stress, which induces a strain wave, i.e., coherent longitudinal acoustic phonon (CLAP), due to thermal expansion caused by the spatial temperature gradient of material [36–39]. The CLAP propagates away from the surface of the material in the direction of the temperature gradient at sound speed, which modifies the local dielectric constant. When the delayed probe light is incident onto the material overlapped with a pump pulse, the transmissivity of probe light will be modified by the local modulated dielectric function thanks to the launch of CLAP. Therefore, the transmission change characterized by periodic oscillation can be sampled [22,40].
Figure 2(d) visualizes the different thermal responses to ultrafast laser irradiation in the thin and thick films, in which the gradient force is essential in driving the generation of CAP [38]. When the film is thin, as in our case of 6.8 nm, the photo-driven thermalization of the film is homogeneous in the propagation direction of pump pulse, as illustrated on the left drawing in Fig. 2(d). The absence of a temperature gradient signifies that the strain wave cannot be delivered, which is why we did not observe the CAP in 6.8 nm
To gain more insight into probing the wavelength-dependent carrier and coherent phonon dynamics of 6.8 and 20 nm
Figure 3.Time- and spectrum-resolved ultrafast TA mapping of (a) 6.8 nm
We first discuss the relaxation of the electronic system under different probe energy. The general profile of photocarriers’ relaxation of 6.8 and 20 nm films is mostly the same. One prominent feature is that the dynamics of the red and blue sides around 650 nm [as the marks of black dash lines in Figs. 3(a) and 3(c)] are distinct. The relaxation of red side, i.e., 650–760 nm, consists of two components: a subpicosecond fast relaxation followed by a slow process with a lifetime of more than 100 ps, which is similar to the experimental results measured with degenerate 780 nm OPOP. By comparison, the relaxation of the blue side of 650 nm shows an extra slow component except for the fast and slow processes. This slow process has a time constant of a few nanoseconds, which reflects the long-lived electronic states at the higher-lying energy band in
The coherent collective excitations with probe wavelength dependence in
Figure 4.Probe wavelength-dependent COP dynamics of
Figure 5.The TA plotting of (a) 6.8 nm and (b) 20 nm
Figure 6.Residual periodical oscillatory signals of 6.8 nm
Figure 7.Damping time of coherent optical phonon of 6.8 nm
In general, two mechanisms have been proposed to explicate the generation of COP excited by ultrafast optical pulse: impulsive stimulated Raman scattering (ISRS) and displacive excitation of coherent phonon (DECP) [43–45]. In the ISRS mechanism, an ultrashort laser pulse (pulse duration must be shorter compared with the oscillatory period of lattice), which has a distribution of frequency containing
Essentially, ultrafast photoexcitation leads to a displacement of the Te atoms along the outward direction, in turn causing a change in the energy band structure, accompanied by a modulation of optical constants. The coherent vibration of the atoms induced by the
4. CONCLUSION
In summary, the ultrafast OPOP measurements were conducted to uncover the photocarriers’ relaxation dynamics and the generation mechanisms of coherent phonon in type-II DSM
APPENDIX A: THE OBSERVATION OF COP IN TA MEASUREMENTS
To more clearly show the damping oscillations induced by
References
[1] Z. K. Liu, B. Zhou, Y. Zhang, Z. J. Wang, H. M. Weng, D. Prabhakaran, M. Z. Mo, Z. X. Shen, Z. Fang, X. Dai, Z. Hussain, Y. L. Chen. Discovery of a three-dimensional topological Dirac semimetal, Na3Bi. Science, 343, 864-867(2014).
[2] S.-Y. Xu, C. Liu, S. K. Kushwaha, R. Sankar, J. W. Krizan, I. Belopolski, M. Neupane, G. Bian, N. Alidoust, T.-R. Chang, H.-T. Jeng, C.-Y. Huang, W.-F. Tsai, H. Lin, P. P. Shibayev, F.-C. Chou, R. J. Cava, M. Z. Hasan. Observation of Fermi arc surface states in a topological metal. Science, 347, 294-298(2015).
[3] Z. K. Liu, J. Jiang, B. Zhou, Z. J. Wang, Y. Zhang, H. M. Weng, D. Prabhakaran, S. K. Mo, H. Peng, P. Dudin, T. Kim, M. Hoesch, Z. Fang, X. Dai, Z. X. Shen, D. L. Feng, Z. Hussain, Y. L. Chen. A stable three-dimensional topological Dirac semimetal Cd3As2. Nat. Mater., 13, 677-681(2014).
[4] S. Borisenko, Q. Gibson, D. Evtushinsky, V. Zabolotnyy, B. Buchner, R. J. Cava. Experimental realization of a three-dimensional Dirac semimetal. Phys. Rev. Lett., 113, 027603(2014).
[5] H.-J. Noh, J. Jeong, E.-J. Cho, K. Kim, B. I. Min, B.-G. Park. Experimental realization of type-II Dirac fermions in a PdTe2 superconductor. Phys. Rev. Lett., 119, 016401(2017).
[6] A. A. Soluyanov, D. Gresch, Z. Wang, Q. Wu, M. Troyer, X. Dai, B. A. Bernevig. Type-II Weyl semimetals. Nature, 527, 495-498(2015).
[7] M. Yan, H. Huang, K. Zhang, E. Wang, W. Yao, K. Deng, G. Wan, H. Zhang, M. Arita, H. Yang, Z. Sun, H. Yao, Y. Wu, S. Fan, W. Duan, S. Zhou. Lorentz-violating type-II Dirac fermions in transition metal dichalcogenide PtTe2. Nat. Commun., 8, 257(2017).
[8] A. Politano, G. Chiarello, B. Ghosh, K. Sadhukhan, C.-N. Kuo, C. S. Lue, V. Pellegrini, A. Agarwal. 3D Dirac plasmons in the type-II Dirac semimetal PtTe2. Phys. Rev. Lett., 121, 086804(2018).
[9] L. Fu, D. Hu, R. G. Mendes, M. H. Rummeli, Q. Dai, B. Wu, L. Fu, Y. Liu. Highly organized epitaxy of Dirac semimetallic PtTe2 crystals with extrahigh conductivity and visible surface plasmons at edges. ACS Nano, 12, 9405-9411(2018).
[10] S. Hao, J. Zeng, T. Xu, X. Cong, C. Wang, C. Wu, Y. Wang, X. Liu, T. Cao, G. Su, L. Jia, Z. Wu, Q. Lin, L. Zhang, S. Yan, M. Guo, Z. Wang, P. Tan, L. Sun, Z. Ni, S.-J. Liang, X. Cui, F. Miao. Low-temperature eutectic synthesis of PtTe2 with weak antilocalization and controlled layer thinning. Adv. Funct. Mater., 28, 1803746(2018).
[11] M. K. Lin, R. A. B. Villaos, J. A. Hlevyack, P. Chen, R. Y. Liu, C. H. Hsu, J. Avila, S. K. Mo, F. C. Chuang, T. C. Chiang. Dimensionality-mediated semimetal-semiconductor transition in ultrathin PtTe2 films. Phys. Rev. Lett., 124, 036402(2020).
[12] K. Deng, M. Yan, C.-P. Yu, J. Li, X. Zhou, K. Zhang, Y. Zhao, K. Miyamoto, T. Okuda, W. Duan, Y. Wu, X. Zhong, S. Zhou. Crossover from 2D metal to 3D Dirac semimetal in metallic PtTe2 films with local Rashba effect. Sci. Bull., 64, 1044-1048(2019).
[13] F. Fei, X. Bo, P. Wang, J. Ying, J. Li, K. Chen, Q. Dai, B. Chen, Z. Sun, M. Zhang, F. Qu, Y. Zhang, Q. Wang, X. Wang, L. Cao, H. Bu, F. Song, X. Wan, B. Wang. Band structure perfection and superconductivity in type-II Dirac semimetal Ir1-
[14] J. Jiang, S. Lee, F. Fei, F. Song, E. Vescovo, K. Kaznatcheev, F. J. Walker, C. H. Ahn. A comprehensive ARPES study on the type-II Dirac semimetal candidate Ir1-
[15] X. W. Tong, Y. N. Lin, R. Huang, Z. X. Zhang, C. Fu, D. Wu, L. B. Luo, Z. J. Li, F. X. Liang, W. Zhang. Direct tellurization of Pt to synthesize 2D PtTe2 for high-performance broadband photodetectors and NIR image sensors. ACS Appl. Mater. Interfaces, 12, 53921-53931(2020).
[16] M. S. Shawkat, S. B. Hafiz, M. M. Islam, S. A. Mofid, M. M. Al Mahfuz, A. Biswas, H. S. Chung, E. Okogbue, T. J. Ko, D. Chanda, T. Roy, D. K. Ko, Y. Jung. Scalable van der Waals two-dimensional PtTe2 layers integrated onto silicon for efficient near-to-mid infrared photodetection. ACS Appl. Mater. Interfaces, 13, 15542-15550(2021).
[17] H. Xu, C. Guo, J. Zhang, W. Guo, C. N. Kuo, C. S. Lue, W. Hu, L. Wang, G. Chen, A. Politano, X. Chen, W. Lu. PtTe2-based type-II Dirac semimetal and its van der Waals heterostructure for sensitive room temperature terahertz photodetection. Small, 15, 1903362(2019).
[18] P. Suo, H. Zhang, S. Yan, W. Zhang, J. Fu, X. Lin, S. Hao, Z. Jin, Y. Zhang, C. Zhang, F. Miao, S.-J. Liang, G. Ma. Observation of negative terahertz photoconductivity in large area type-II Dirac semimetal PtTe2. Phys. Rev. Lett., 126, 227402(2021).
[19] N. Kumar, B. A. Ruzicka, N. P. Butch, P. Syers, K. Kirshenbaum, J. Paglione, H. Zhao. Spatially resolved femtosecond pump-probe study of topological insulator Bi2Se3. Phys. Rev. B, 83, 235306(2011).
[20] A. Othonos. Probing ultrafast carrier and phonon dynamics in semiconductors. J. Appl. Phys., 83, 1789-1830(1998).
[21] J. Fu, M. Jiang, P. Suo, W. Zhang, X. Lin, X. Yan, S. Zhang, G. Ma. Optically controlled ultrafast terahertz switching in wafer scale PtSe2 thin films. Appl. Opt., 60, 5037-5043(2021).
[22] D. Li, J. Fu, P. Suo, W. Zhang, B. Lu, X. Lin, X. Yan, B. Li, G. Ma, J. Yao. Layer dependent interlayer coherent phonon dynamics in PdSe2 films. Appl. Phys. Lett., 118, 191105(2021).
[23] J. Wei, Y. Wu, R. Pu, L. Shi, J. Jiang, J. Du, Z. Guo, Y. Huang, W. Liu. Tracking ultrafast structural dynamics in a dual-emission anti-kasha-active fluorophore using femtosecond stimulated Raman spectroscopy. J. Phys. Chem. Lett., 12, 4466-4473(2021).
[24] J. Fu, W. Xu, X. Chen, S. Zhang, W. Zhang, P. Suo, X. Lin, J. Wang, Z. Jin, W. Liu, G. Ma. Thickness-dependent ultrafast photocarrier dynamics in selenizing platinum thin films. J. Phys. Chem. C, 124, 10719-10726(2020).
[25] T. C. Sum, N. Mathews, G. Xing, S. S. Lim, W. K. Chong, D. Giovanni, H. A. Dewi. Spectral features and charge dynamics of lead halide perovskites: origins and interpretations. Acc. Chem. Res., 49, 294-302(2016).
[26] M. Breusing, C. Ropers, T. Elsaesser. Ultrafast carrier dynamics in graphite. Phys. Rev. Lett., 102, 086809(2009).
[27] C. Zhu, X. Yuan, F. Xiu, C. Zhang, Y. Xu, R. Zhang, Y. Shi, F. Wang. Broadband hot carrier dynamics in three-dimensional Dirac semimetal Cd3As2. Appl. Phys. Lett., 111, 091101(2017).
[28] P. A. George, J. Strait, J. Dawlaty, S. Shivaraman, M. Chandrashekhar, F. Rana, M. G. Spencer. Ultrafast optical-pump terahertz-probe spectroscopy of the carrier relaxation and recombination dynamics in epitaxial graphene. Nano Lett., 8, 4248-4251(2008).
[29] G. Wang, K. Wang, N. McEvoy, Z. Bai, C. P. Cullen, C. N. Murphy, J. B. McManus, J. J. Magan, C. M. Smith, G. S. Duesberg, I. Kaminer, J. Wang, W. J. Blau. Ultrafast carrier dynamics and bandgap renormalization in layered PtSe2. Small, 15, 1902728(2019).
[30] H. Haug, S. W. Koch. Quantum Theory of the Optical and Electronic Properties of Semiconductors(2009).
[31] R. P. Prasankumar, A. J. Taylor. Optical Techniques for Solid-State Materials Characterization(2016).
[32] W. Lu, S. Ge, X. Liu, H. Lu, C. Li, J. Lai, C. Zhao, Z. Liao, S. Jia, D. Sun. Ultrafast relaxation dynamics of photoexcited Dirac fermions in the three-dimensional Dirac semimetal Cd3As2. Phys. Rev. B, 95, 024303(2017).
[33] Y. M. Dai, J. Bowlan, H. Li, H. Miao, S. F. Wu, W. D. Kong, Y. G. Shi, S. A. Trugman, J. X. Zhu, H. Ding, A. J. Taylor, D. A. Yarotski, R. P. Prasankumar. Ultrafast carrier dynamics in the large-magnetoresistance material WTe2. Phys. Rev. B, 92, 161104(2015).
[34] S.-X. Zhu, C. Zhang, Q.-Y. Wu, X.-F. Tang, H. Liu, Z.-T. Liu, Y. Luo, J.-J. Song, F.-Y. Wu, Y.-Z. Zhao, S.-Y. Liu, T. Le, X. Lu, H. Ma, K.-H. Liu, Y.-H. Yuan, H. Huang, J. He, H. Y. Liu, Y.-X. Duan, J.-Q. Meng. Temperature evolution of quasiparticle dispersion and dynamics in semimetallic 1T-TiTe2 via high-resolution angle-resolved photoemission spectroscopy and ultrafast optical pump-probe spectroscopy. Phys. Rev. B, 103, 115108(2021).
[35] Y. M. Sheu, Y. J. Chien, C. Uher, S. Fahy, D. A. Reis. Free-carrier relaxation and lattice heating in photoexcited bismuth. Phys. Rev. B, 87, 075429(2013).
[36] C. Thomsen, J. Strait, Z. Vardeny, H. J. Maris, J. Tauc, J. J. Hauser. Coherent phonon generation and detection by picosecond light pulses. Phys. Rev. Lett., 53, 989-992(1984).
[37] C. Thomsen, H. T. Grahn, H. J. Maris, J. Tauc. Surface generation and detection of phonons by picosecond light pulses. Phys. Rev. B, 34, 4129-4138(1986).
[38] Y. Wang, L. Guo, X. Xu, J. Pierce, R. Venkatasubramanian. Origin of coherent phonons in Bi2Te3 excited by ultrafast laser pulses. Phys. Rev. B, 88, 064307(2013).
[39] X. Chen, S. Zhang, L. Wang, Y.-F. Huang, H. Liu, J. Huang, N. Dong, W. Liu, I. M. Kislyakov, J. M. Nunzi, L. Zhang, J. Wang. Direct observation of interlayer coherent acoustic phonon dynamics in bilayer and few-layer PtSe2. Photon. Res., 7, 1416-1424(2019).
[40] S. Ge, X. Liu, X. Qiao, Q. Wang, Z. Xu, J. Qiu, P. H. Tan, J. Zhao, D. Sun. Coherent longitudinal acoustic phonon approaching THz frequency in multilayer molybdenum disulphide. Sci. Rep., 4, 5722(2014).
[41] S. Soled, A. Wold, O. Gorochov. Crystal growth and characterization of platinum ditelluride. Mat. Res. Bull., 10, 831-836(1975).
[42] F. Vialla, N. D. Fatti. Time-domain investigations of coherent phonons in van der Waals thin films. Nanomaterials, 10, 2543(2020).
[43] K. J. Yee, Y. S. Lim, T. Dekorsy, D. S. Kim. Mechanisms for the generation of coherent longitudinal-optical phonons in GaAs/AlGaAs multiple quantum wells. Phys. Rev. Lett., 86, 1630-1633(2001).
[44] A. R. Attar, H. T. Chang, A. Britz, X. Zhang, M. F. Lin, A. Krishnamoorthy, T. Linker, D. Fritz, D. M. Neumark, R. K. Kalia, A. Nakano, P. Ajayan, P. Vashishta, U. Bergmann, S. R. Leone. Simultaneous observation of carrier-specific redistribution and coherent lattice dynamics in 2H-MoTe2 with femtosecond core-level spectroscopy. ACS Nano, 14, 15829-15840(2020).
[45] J. Fu, M. Li, A. Solanki, Q. Xu, Y. Lekina, S. Ramesh, Z. X. Shen, T. C. Sum. Electronic states modulation by coherent optical phonons in 2D halide perovskites. Adv. Mater., 33, 2006233(2021).
[46] Y.-X. Yan, E. B. Gamble, K. Nelson. Impulsive stimulated scattering: general importance in femtosecond laser pulse interactions with matter, and spectroscopic applications. J. Chem. Phys., 83, 5391-5399(1985).
[47] S. D. Silvestri, J. G. Fujimoto, E. P. Ippen, E. B. Bamble, L. R. Williams, K. A. Nelson. Femtosecond time-resolved measurements of optic phonon dephasing by impulsive stimulated Raman scattering in α-perylene crystal from 20 to 300 K. Chem. Phys. Lett., 116, 146-152(1985).
[48] A. M. Weiner, D. E. Leaird, G. P. Wiederrecht, K. Nelson. Femtosecond pulse sequences used for optical manipulation of molecular motion. Science, 247, 1317-1319(1990).
[49] G. C. Cho, W. Kutt, H. Kurz. Subpicosecond time-resolved coherent-phonon oscillations in GaAs. Phys. Rev. Lett., 65, 764-766(1990).
[50] L. Dhar, J. A. Rogers, K. A. Nelson. Time-resolved vibrational spectroscopy in the impulsive limit. Chem. Rev., 94, 157-193(1994).
[51] T. K. Cheng, J. Vidal, H. J. Zeiger, G. Dresselhaus, M. S. Dresselhaus, E. P. Ippen. Mechanism for displacive excitation of coherent phonons in Sb, Bi, Te, and Ti2O3. Appl. Phys. Lett., 59, 1923-1925(1991).
[52] H. J. Zeiger, J. Vidal, T. K. Cheng, E. P. Ippen, G. Dresselhaus, M. S. Dresselhaus. Theory for displacive excitation of coherent phonons. Phys. Rev. B, 45, 768-778(1992).
[53] G. A. Garrett, T. F. Albrecht, J. F. Whitaker, R. Merlin. Coherent THz phonons driven by light pulses and the Sb problem: what is the mechanism?. Phys. Rev. Lett., 77, 3661-3664(1996).
[54] C. Trovatello, H. P. C. Miranda, A. Molina-Sanchez, R. Borrego-Varillas, C. Manzoni, L. Moretti, L. Ganzer, M. Maiuri, J. Wang, D. Dumcenco, A. Kis, L. Wirtz, A. Marini, G. Soavi, A. C. Ferrari, G. Cerullo, D. Sangalli, S. D. Conte. Strongly coupled coherent phonons in single-layer MoS2. ACS Nano, 14, 5700-5710(2020).
[55] T. Y. Jeong, B. M. Jin, S. H. Rhim, L. Debbichi, J. Park, Y. D. Jang, H. R. Lee, D. H. Chae, D. Lee, Y. H. Kim, S. Jung, K. J. Yee. Coherent lattice vibrations in mono- and few-layer WSe2. ACS Nano, 10, 5560-5566(2016).
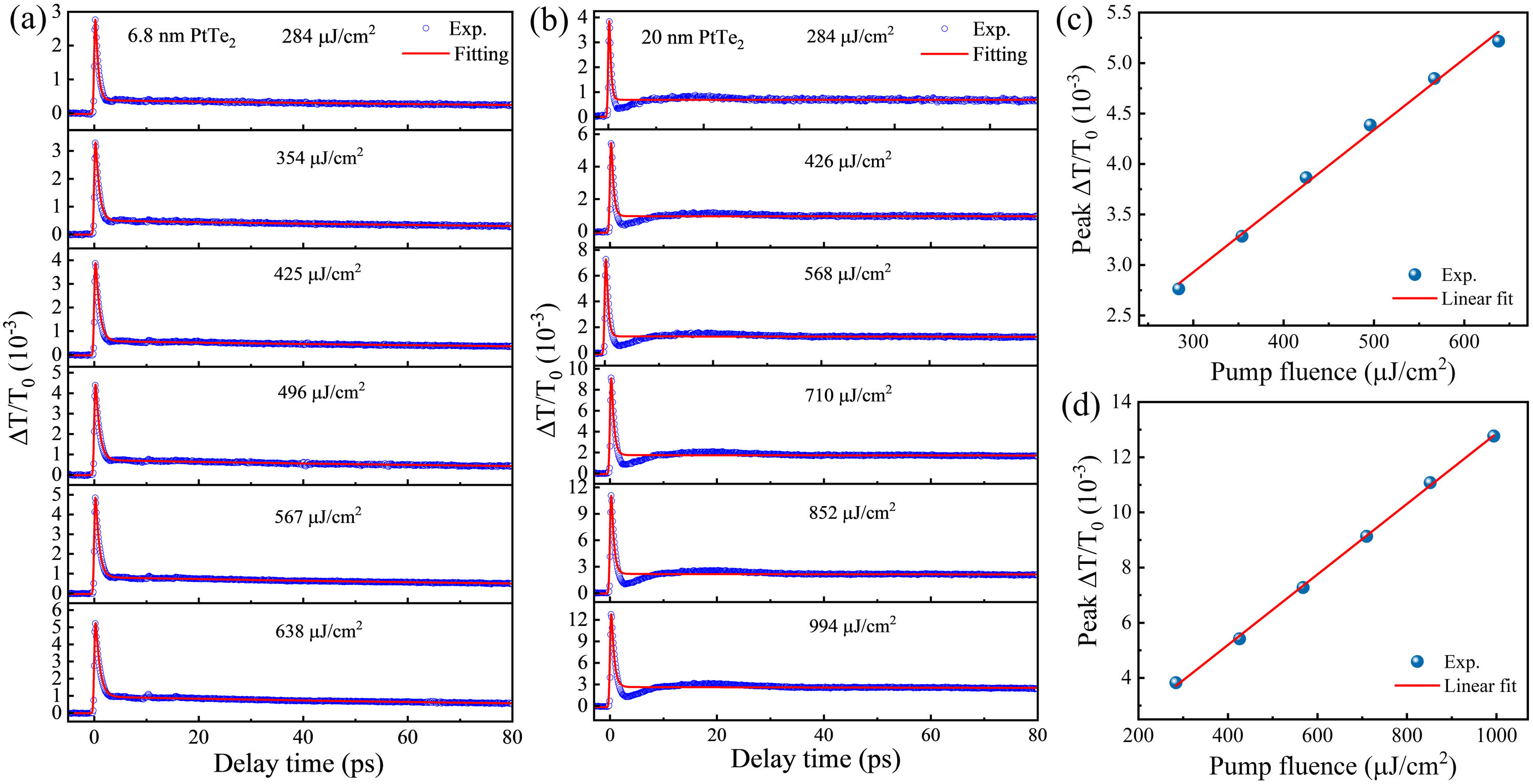
Set citation alerts for the article
Please enter your email address