
- Chinese Optics Letters
- Vol. 19, Issue 11, 111401 (2021)
Abstract
1. Introduction
Chaotic lasers have attracted a lot of attention and received intensive investigation for their applications in random bit generation[
Several approaches utilizing two or more lasers have been proposed to break through the limitation of relaxation-oscillation frequency and enhance the chaotic bandwidth, e.g., injecting chaotic signals from one laser to another laser[
In this Letter, we propose a wideband chaos generation system with a single-cavity dual-mode microsquare laser. The intensity ratio between the dual lasing modes can be tuned by adjusting the biased current of the microsquare laser. Based on the dual-mode microsquare laser with balanced intensity, wideband chaos with a standard bandwidth of 31.3 GHz is realized by tuning the feedback strength. Benefiting from the simple structure of the dual-mode laser, the system is easy to implement without tuning multiple currents.
Sign up for Chinese Optics Letters TOC. Get the latest issue of Chinese Optics Letters delivered right to you!Sign up now
2. Experimental Setup
Figure 1 shows the experimental setup for wideband chaotic signal generation. The dual-mode microsquare semiconductor laser is mounted on a thermoelectric cooler (TEC) to fix the working temperature. The output light from the laser is collected by a single-mode fiber (SMF) and then coupled into a feedback loop with a length of 51.4 m. The feedback loop contains an optical circulator (OC) to guide the light out and into the laser, an erbium-doped fiber amplifier (EDFA) with maximum output power of 10 dBm, and an optical bandpass filter (OBPF) to amplify and filter out the optical signal at the target wavelength, as shown in Fig. 2(d). The OBPF (BVF-200CL, Alnair Labs) has a tunable bandwidth of 0.1–13 nm and an insertion loss of 3.5 dB. Besides, a polarization controller (PC) is utilized to control the polarization. The optical signal is divided into two equal parts by a 50/50 optical splitter. One part is for the feedback loop, and the other part is converted to an electric signal by a high-speed photodetector (PD) [XPDV21x0(RA)]. The electric RF signal is then measured by a 50 GHz electric spectrum analyzer (ESA) (R&S FSWP50).
Figure 1.Experimental setup for wideband chaotic signal generation based on dual-mode microsquare laser. OC, optical circulator; EDFA, erbium-doped fiber amplifier; OBPF, optical bandpass filter; PD, photodetector; PC, polarization controller; ESA, electric spectrum analyzer.
Figure 2.(a) Microscopic image of the microsquare laser. (b) Representative lasing spectra of the microsquare laser. (c) Magnetic field distributions of the fundamental (zeroth-order) and first-order (1st-order) mode. (d) Lasing spectrum at 50 mA in a large scale to show the longitudinal mode characteristics and the function of the OBPF.
3. Results
Figure 2(a) shows the microscopic image of a microsquare semiconductor laser composed of a square microcavity with an output waveguide[
The SMF coupled optical power from the laser is about 70 µW at 50 mA, and an EDFA is used to amplify the feedback optical power. The feedback strength is defined as the ratio of the optical power entering port 1 of the OC to the output power of the laser. The measured RF spectra at different currents are presented in Fig. 3 at the feedback strength of 7.3 dB. At 31 mA, the RF spectrum shows a broad peak at about 8.9 GHz related to the relaxation-oscillation frequency of the microsquare laser. Because the two transverse modes have a large intensity ratio of 39.3 dB, the RF spectra are similar to that measured with a single-mode laser. With the increase of biased current, the intensity of the mode at the longer-wavelength side increases, as shown in Fig. 2(b), and, hence, the peak at the frequency of
Figure 3.RF spectra of the generated chaotic signal at different currents with fixed feedback strength of 7.3 dB.
Figure 4.Intensity ratio between two lasing modes (blue squares) and standard bandwidth (red circles) of the chaotic signal versus the biased currents. Inset: flatness of the RF spectrum versus biased current.
The standard bandwidths of the chaotic signal versus the biased currents are plotted in Fig. 4 as red circles. The standard bandwidth is defined as the range between the DC and the frequency that contains 80% of the RF spectral power[
At a biased current of 51 mA, optical spectra are measured under different feedback strengths and plotted in Fig. 5, which are vertically shifted by 5 dB for clarity. When the feedback strength decreases from 7.3 dB to 3.8 dB by adjusting the amplification rate of the EDFA, the
Figure 5.Lasing spectra of the microsquare laser with a fixed biased current of 51 mA and different feedback strengths.
The RF spectra with different feedback strengths are shown in Fig. 6(a). As the feedback strength decreases from 7.3 to 0.8 dB, both the mode-beating peak and the relaxation-oscillation peak become narrow[
Figure 6.(a) Representative RF spectra with different feedback strengths at 51 mA. (b) Flatness in the standard bandwidth, standard bandwidth, and effective bandwidth as a function of the feedback strength, indicated by symbols of red circles, blue squares, and blue triangles.
Besides, the RF spectral level decreases with the feedback strength decrease, which is because the detuning of the feedback strength by EDFA also reduces the optical power into the PD.
The above results show that wideband chaos can be generated based on the dual-mode microsquare laser. A flat RF spectrum can be obtained with balanced dual-mode lasing and high feedback strength. To further improve the flatness, we replace the 50/50 optical splitter by an 80/20 one with the 80% power feedback to the laser to increase the feedback strength (10.3 dB). A flatter chaotic signal covering more than 50 GHz in the spectrum is obtained as shown in Fig. 7(a). The spectrum is distinctly higher than the noise floor, which is typical for chaotic signals. The standard bandwidth of the RF spectrum is 31.3 GHz with a flatness of 8.3 dB. The effective bandwidth of the RF spectrum is 30.7 GHz with a flatness of 6.1 dB. Besides, the low frequency components rise because of the competition of the modes[
Figure 7.(a) RF spectrum with feedback strength of 10.3 dB at 51 mA. (b) Time series and (c) autocorrelation coefficient of the chaotic signal.
The microsquare laser is designed for dual-mode lasing with a mode interval of about 30 GHz[
4. Conclusion
In conclusion, we have proposed and demonstrated the generation of wideband chaotic signals based on a dual-mode microsquare laser under optical feedback. By adjusting the feedback strength to 10.3 dB and the intensity ratio of the two modes to 0 dB, a wideband chaotic signal covering more than 50 GHz in the RF spectrum can be achieved. The standard bandwidth and the effective bandwidth of the chaotic signal are 31.3 GHz and 30.7 GHz with flatness of 8.3 dB and 6.1 dB, respectively. The chaotic signal with wide bandwidth and good flatness, generated from a single-cavity dual-mode laser with optical feedback, demands a good match of the dual-mode intensity ratio and feedback strength. The principle also implies that chaotic signals with higher bandwidth and better flatness can be achieved by utilizing a three or multimode laser with optical feedback, which is important for better applications of chaotic lasers.
References
[1] M. Sciamanna, K. A. Shore. Physics and applications of laser diode chaos. Nat. Photon., 9, 151(2015).
[2] A. Uchida, K. Amano, M. Inoue, K. Hirano, S. Naito, H. Someya, I. Oowada, T. Kurashige, M. Shiki, S. Yoshimori, K. Yoshimura, P. Davis. Fast physical random bit generation with chaotic semiconductor lasers. Nat. Photon., 2, 728(2008).
[3] P. Li, Y. Guo, Y. Guo, Y. Fan, X. Guo, X. Liu, K. Li, K. A. Shore, Y. Wang, A. Wang. Ultrafast fully photonic random bit generator. J. Lightwave Technol., 36, 2531(2018).
[4] M. Li, Y. Chen, Y. Song, C. Zeng, X. Zhang. DOE effect on BER performance in MSK space uplink chaotic optical communication. Chin. Opt. Lett., 18, 070601(2020).
[5] J. Ke, L. Yi, G. Xia, W. Hu. Chaotic optical communications over 100-km fiber transmission at 30-Gb/s bit rate. Opt. Lett., 43, 1323(2018).
[6] X. Wang, S. Li, X. Jiang, J. Hu, M. Xue, S. Xu, S. Pan. High-accuracy optical time delay measurement in fiber link. Chin. Opt. Lett., 17, 060601(2019).
[7] Z. Hu, B. Wang, L. Wang, T. Zhao, H. Han, Y. Wang, A. Wang. Improving spatial resolution of chaos OTDR using significant-bit correlation detection. IEEE Photon. Technol. Lett., 31, 1029(2019).
[8] F. Y. Lin, J. M. Liu. Chaotic lidar. IEEE J. Sel. Top. Quantum Electron., 10, 991(2004).
[9] Y. Xiao, T. Deng, Z.-M. Wu, J.-G. Wu, X.-D. Lin, X. Tang, L.-B. Zeng, G.-Q. Xia. Chaos synchronization between arbitrary two response VCSELs in a broadband chaos network driven by a bandwidth-enhanced chaotic signal. Opt. Commun., 285, 1442(2012).
[10] I. Reidler, Y. Aviad, M. Rosenbluh, I. Kanter. Ultrahigh-speed random number generation based on a chaotic semiconductor laser. Phys. Rev. Lett., 103, 024102(2009).
[11] I. Kanter, Y. Aviad, I. Reidler, E. Cohen, M. Rosenbluh. An optical ultrafast random bit generator. Nat. Photon., 4, 58(2010).
[12] K. Hirano, T. Yamazaki, S. Morikatsu, H. Okumura, H. Aida, A. Uchida, S. Yoshimori, K. Yoshimura, T. Harayama, P. Davis. Fast random bit generation with bandwidth-enhanced chaos in semiconductor lasers. Opt. Express, 18, 5512(2010).
[13] Y. Wang, Z. Jia, Z. Gao, J. Xiao, L. Wang, Y. Wang, Y. Huang, A. Wang. Generation of laser chaos with wide-band flat power spectrum in a circular-side hexagonal resonator microlaser with optical feedback. Opt. Express, 28, 18507(2020).
[14] R. Sakuraba, K. Iwakawa, K. Kanno, A. Uchida. Tb/s physical random bit generation with bandwidth-enhanced chaos in three-cascaded semiconductor lasers. Opt. Express, 23, 1470(2015).
[15] L. Qiao, T. Lv, Y. Xu, M. Zhang, J. Zhang, T. Wang, R. Zhou, Q. Wang, H. Xu. Generation of flat wideband chaos based on mutual injection of semiconductor lasers. Opt. Lett., 44, 5394(2019).
[16] B. Pan, D. Lu, L. Zhao. Broadband chaos generation using monolithic dual-mode laser with optical feedback. IEEE Photon. Technol. Lett., 27, 2516(2015).
[17] Q. Yang, L. Qiao, M. Zhang, J. Zhang, T. Wang, S. Gao, M. Chai, P. M. Mohiuddin. Generation of a broadband chaotic laser by active optical feedback loop combined with a high nonlinear fiber. Opt. Lett., 45, 1750(2020).
[18] H. Long, Y.-Z. Huang, X.-W. Ma, Y.-D. Yang, J.-L. Xiao, L.-X. Zou, B.-W. Liu. Dual-transverse-mode microsquare lasers with tunable wavelength interval. Opt. Lett., 40, 3548(2015).
[19] Y.-D. Yang, Y.-Z. Huang. Mode characteristics and directional emission for square microcavity lasers. J. Phys. D, 49, 253001(2016).
[20] H.-Z. Weng, Y.-Z. Huang, X.-W. Ma, F.-L. Wang, M.-L. Liao, Y.-D. Yang, J.-L. Xiao. Spectral linewidth analysis for square microlasers. IEEE Photon. Technol. Lett., 29, 1931(2017).
[21] H.-Z. Weng, Y.-D. Yang, J.-L. Wu, Y.-Z. Hao, M. Tang, J.-L. Xiao, Y.-Z. Huang. Dual-mode microcavity semiconductor lasers. IEEE J. Sel. Top. Quantum Electron., 25, 1501408(2019).
[22] H. Someya, I. Oowada, H. Okumura, T. Kida, A. Uchida. Synchronization of bandwidth-enhanced chaos in semiconductor lasers with optical feedback and injection. Opt. Express, 17, 19536(2009).
[23] R. Takahashi, Y. Akizawa, A. Uchida, T. Harayama, K. Tsuzuki, S. Sunada, K. Arai, K. Yoshimura, P. Davis. Fast physical random bit generation with photonic integrated circuits with different external cavity lengths for chaos generation. Opt. Express, 22, 11727(2014).
[24] B. Tromborg, H. Olesen, X. Pan, S. Saito. Transmission line description of optical feedback and injection locking for Fabry–Perot and DFB lasers. IEEE J. Sel. Top. Quantum Electron., 23, 1875(1987).
[25] F.-Y. Lin, Y.-K. Chao, T.-C. Wu. Effective bandwidths of broadband chaotic signals. IEEE. J. Quantum Electron., 48, 1010(2012).
[26] P. Li, Q. Cai, J. Zhang, B. Xu, Y. Liu, A. Bogris, K. A. Shore, Y. Wang. Observation of flat chaos generation using an optical feedback multi-mode laser with a band-pass filter. Opt. Express, 27, 17859(2019).
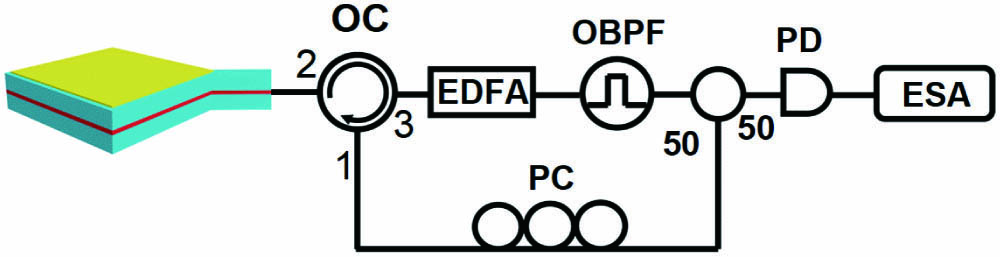
Set citation alerts for the article
Please enter your email address