Abstract
The denaturation of double-stranded deoxyribonucleic acid (ds-DNA) has been well known to break nucleobase bonds, resulting in single-stranded deoxyribonucleic acid (ss-DNA) in solutions, which can recombine to form ds-DNA in a reversible manner. We developed an efficient process to irreversibly maintain various DNA denaturation levels in thin solid films in order to investigate the impacts of the denaturation on the optical properties of DNA films. By adding NaOH in an aqueous solution of salmon testis DNA, we flexibly controlled the level of denaturation in the solution, which was then spin-coated on Si and silica substrates to irreversibly bind ss-DNAs in a thin solid film. The denaturation of DNA in thin solid films was experimentally confirmed by ultraviolet-visible and Fourier transform infrared spectroscopic investigations, whose level could be controlled by the NaOH content in the aqueous solution precursor. By this irreversible denaturation process, we developed a new method to flexibly vary the refractive index of DNA thin solid films in a wide range of in the visible to near-infrared range. Thermo-optic coefficients dn/dT of the films were also experimentally measured in the temperature range from 40°C to 90°C to confirm the significant impacts of denaturation. Detailed thin film processes and optical characterizations are discussed.1. INTRODUCTION
Since the discovery of the deoxyribonucleic acid (DNA) double-helix structure [1], DNA has been incessantly investigated in various areas of life sciences, biomedical technologies, and recently, physical sciences. DNA thin solid films (TSFs) have drawn intense attention due to their unique potential as a highly functional layer in electronic devices [2,3] and organic light-emitting diode (OLED) devices [4]. In photonic devices, DNA-TSF layers have been also employed successfully, such as an active layer in an optical amplifier [5], a functional cladding layer in an electro-optic modulator [6], a saturable absorber in pulse generation [7], and in various optical sensors [8,9] to name a few. However, these prior DNA applications have been limited to hybrid structures such that DNA-TSFs have been embedded in device platforms made of inorganic or other types of polymer materials. In order to further pursue all-DNA photonic waveguide devices fully utilizing the inherently biocompatible nature of DNA, systematic, precise, and repeatable control of the refractive index in DNA-TSFs is imperative. The authors have recently shown the potential to control the refractive index of lipid-bound DNA films by developing a re-crystallization process [10]. The method used organic solvents to dissolve the prepared DNA-lipid precipitates, but the lipids bound in DNA films may not fully guarantee the inherent biocompatibility of pure DNA. There have been reports of changing the refractive index of lipid-bound DNA films by adding organic dyes [11], which might further deteriorate biocompatibility. Therefore, it is highly desirable to maintain the full biocompatibility of DNA by removing the lipid complex to use aqueous solution precursors in the refractive index control of DNA-TSFs. The authors have proposed adding vitamin B2 to lipid-free DNA-TSFs to achieve efficient refractive index control [12].
However, this method is based on the additional optical loss of vitamin B2 in the visible range so that the method is applicable for devices operating only in the infrared (IR) region. It is, therefore, very important and useful to maintain the fully biocompatible nature of lipid-free DNA-TSFs while making them operate in a wide spectral range covering both the visible and IR for practical photonic devices.
It has been well understood that double-stranded DNA (ds-DNA) can be separated into single-stranded DNA (ss-DNA), which is called DNA denaturation, by radiation, heat, or using chemical agents in the solution states [13–15]. The impacts of DNA denaturation on both chemical and biological aspects have been well investigated [16,17], but systematic investigation of its influences on the optical perspective has not been attempted, especially for DNA in thin solid film states.
Sign up for Photonics Research TOC. Get the latest issue of Photonics Research delivered right to you!Sign up now
In this study, we developed a novel process to control the optical properties of lipid-free DNA-TSFs, enabling photonic devices operating in a wide spectral range including both the visible and IR regions, utilizing denaturation of ds-DNA into ss-DNA in aqueous solutions and irreversibly maintaining the denaturation level in thin solid films, for the first time to the best knowledge of the authors. It has been reported in prior studies that the level of DNA denaturation in the aqueous solution can be flexibly controlled by adding NaOH and by increasing the pH level [18–21]. However, the denaturation in an aqueous solution has been also known to be chemically reversible, such that ss-DNA can be self-assembled to ds-DNA through a statistical DNA hybridization process in water [22,23]. The notions of this study are: (1) the level of DNA denaturation in an aqueous solution is controlled by adding NaOH; (2) these solution precursors are spin-coated on substrates to form DNA-TSF to irreversibly maintain the DNA denaturation level in the solid state; (3) denaturation changes the density of DNA-TSFs and, subsequently, their refractive indices and thermo-optic properties, in a controllable manner over a wide spectral range. Here, we report highly precise and repeatable control of the refractive index of DNA-TSFs by varying the level of denaturation in a solid, as schematically shown in Fig. 1. We successfully obtained a very wide range of refractive index variation in lipid-free DNA-TSFs, which is sufficiently large to provide all-DNA optical waveguide structures with full biocompatibility operating in a broad spectral band. In the following sections, we reported the spectroscopic identification of DNA denaturation and its correlation with the optical dispersion characteristics and their temperature dependence, which can open a new avenue of all-DNA broadband photonic device applications.
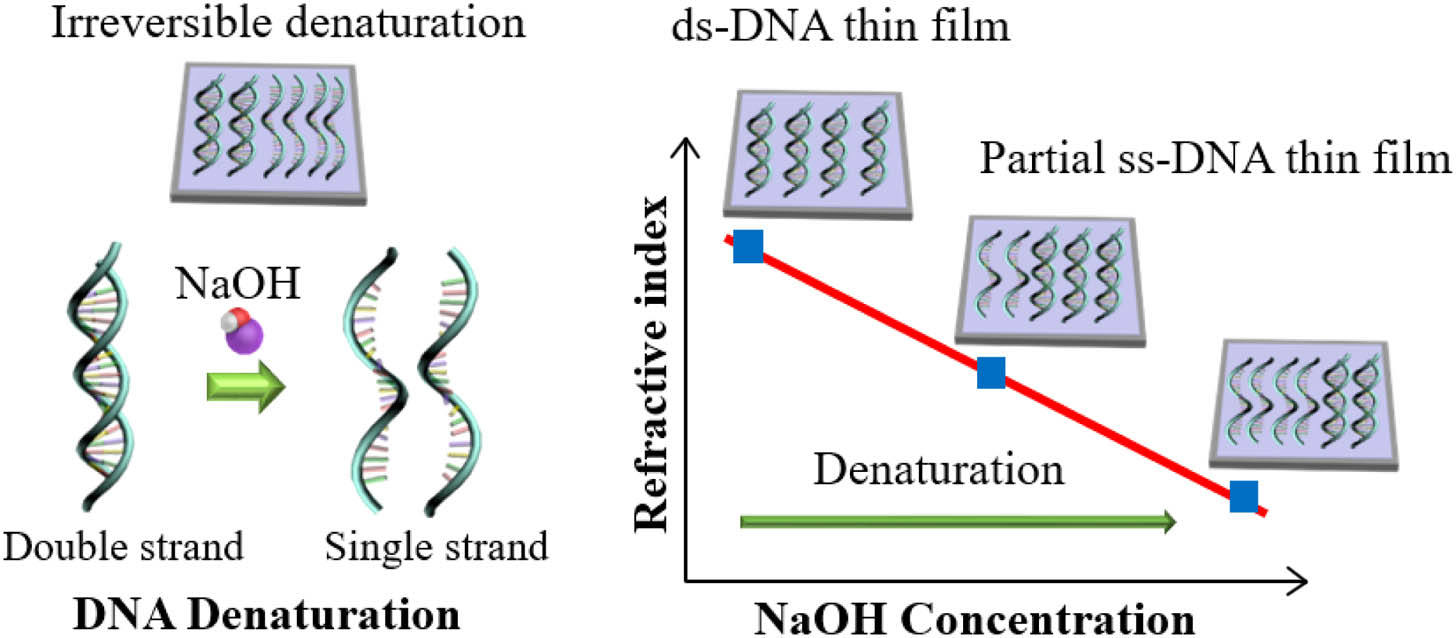
Figure 1.Schematic diagram to control the refractive index of DNA thin solid film by denaturation. Denaturation is activated by adding NaOH in DNA aqueous solution precursors, which is irreversibly immobilized in thin solid film to change the refractive index (ds, double stranded; ss, single stranded).
2. EXPERIMENT
We used salmon testis DNA powder purchased from Ogata Research Laboratories Ltd. in Japan, which has been widely used in DNA-TSF fabrication [7,10,24,25]. The DNA powder was dissolved in deionized water to make homogeneous DNA aqueous solutions with a concentration of 0.4 wt. %. In order to initiate the chemical denaturation in DNA aqueous solutions with a volume of 15 mL, we further dissolved high-purity NaOH to make four distinctive solutions with 0, 2.5, 5.0, and 7.5 mM (1 mM = 1 mmol/L) NaOH concentrations. These NaOH concentration ranges have been known to partially denaturate ds-DNA to result in ss-DNA in aqueous solutions [18,19]. We used these DNA-NaOH solutions as the precursors of DNA thin solid films in order to solidify the ss-DNAs within the ds-DNAs in an irreversible manner, for the first time to our knowledge.
The prepared DNA-NaOH aqueous solution precursors were spin-coated on Si and substrates at 20°C, similar to the previous ds-DNA thin film fabrication processes [7,24]. The processes are schematically shown in Fig. 2. Oxygen plasma treated P-type Si(100) and quartz wafers were used after a conventional ultrasonic cleaning procedure: 5 min in acetone and 5 min in isopropyl alcohol. Over the prepared substrates of a size of , DNA-NaOH aqueous solution precursors of were dropped using a dispenser, which was then spun at 750–800 r/min in a commercial spin coater (ACE-200) for 5 min. These samples were vacuum-dried in a vacuum desiccator at 20°C for 24 h to obtain uniform thin films with a thickness of . Considering both prior reports and our own experiments, it is understood that NaOH in the aqueous solution fundamentally induced the denaturation similar to that in prior reports, and the solution served as a stable liquid precursor for ss-DNA thin solid films. The spin-coating mechanism, where a thin layer of liquid is evaporated within a few seconds, might have corroborated the fixture of denatured ss-DNA in the thin solid film. The fast evaporation in the spin-coating process might have reduced the renaturation rate, effectively maintaining the denaturation state in the thin solid DNA film.
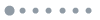
Figure 2.DNA thin solid film fabrication process. (a) DNA aqueous solution and DNA-NaOH aqueous solution where the denaturation is reversible. (b) plasma treatment on substrate to make hydrophilic surfaces. (c) Dispensing aqueous solution precursors on the substrate. (d) Spinning and solidification by water evaporation. (e) Single-stranded DNAs are maintained in the thin solid film to achieve the irreversible denaturation.
Note that in a solution state, ss-DNA can be self-assembled into ds-DNA in a reversible manner. In the case of monolayers, ss-DNA monolayers deposited on various substrates could be hybridized with nanoparticles modified with a complementary ss-DNA to form a ds-DNA monolayer [26,27]. However, we succeeded in irreversibly maintaining ss-DNA within DNA thin multiple layer films fabricated by the spin-coating process.
3. RESULTS AND DISCUSSION
A. UV-Visible Spectrometer to Confirm Denaturation in Aqueous Solutions
In order to confirm the DNA denaturation in the prepared aqueous solution precursors, we measured their UV-visible absorption spectra using a commercial spectrometer (V-650, JASCO Corporation). DNA absorbs the UV light due to the electronic structure of the heterocyclic rings of the nucleotides, and the characteristic absorption of DNA in the aqueous solution occurs near the wavelength [28–30]. It has been widely accepted that the DNA denaturation changes the UV absorption peak intensity [13,18,21], which has been widely utilized as a denaturation signature. In order to clearly resolve the changes in the 260 nm absorption peak, we reduced the concentration of DNA in the aqueous solution to 0.15 wt. % and varied the NaOH concentration from 0 to 17.5 mM. For each NaOH-DNA solution, the UV-visible absorbance was measured, and the results are summarized in Fig. 3.
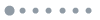
Figure 3.(a) UV/visible spectra of DNA aqueous solution with various NaOH concentrations in the precursor solutions. Here we used 0.15 wt. % DNA. (b) Hyperchromicity near was clearly observed by increasing the NaOH concentration.
When a double-stranded DNA helix unwinds into single strands to form random coils, it is well known that an increase in the absorbance at is observed, which is called the hyperchromicity of DNA denaturation [30]. Consistent with prior reports, we also observed hyperchromicity at as the NaOH concentration increased in the salmon testis DNA aqueous solution, which is the direct evidence of denaturation. We also measured the absorbance of the NaOH aqueous solutions without DNA and we could not find any changes in comparison to pure deionized water in the UV region near , which confirmed that the hyperchromicity of the NaOH-DNA solutions around is attributed only to DNA denaturation. Note that peak absorbance around gradually increased up to the NaOH concentration of 10.0 mM, and it abruptly further increased at a higher concentration. The absorbance around saturated beyond the NaOH concentration of 17.5 mM, and we also observed that the peak position of the absorbance slightly moved toward a longer wavelength as the NaOH concentration increased. In prior reports, how this hyperchromicity is correlated with the exact level of denaturation has not been fully quantified yet. In this study, we will focus on the impacts of the DNA denaturation on the optical properties in the DNA thin solid films. The experimental data will be referenced to the NaOH concentration in the DNA precursor solutions.
B. FTIR Measurement to Confirm Denaturation in Thin Solid Film
We further investigated the molecular structure of solidified NaOH-DNA precursors in a thin film using Fourier transform infrared (FTIR) spectroscopy in the spectral range from 700 to , and the results are summarized in Fig. 4. Here, we used a 0.4 wt. % aqueous solution of DNA with various NaOH concentrations as a solution precursor, which was dropped onto a petri dish and dried in a vacuum desiccator. Freestanding DNA films with a thickness of were obtained with various NaOH concentrations. Figure 4(a) shows the FTIR absorption spectra of the freestanding solid DNA films with various NaOH concentrations in the solution precursors. The FTIR absorption spectra were divided into three spectral regions: for OH stretching, for nucleobase vibrations, and for the sugar and phosphate backbone groups [31]. Since the denaturation process breaks the hydrogen bonds between nucleobases, we focused on how one of the nucleobases, cytosine, would change in its characteristic vibrational peaks for various NaOH concentrations. It is well known that in pristine DNA the cytosine in-plane vibration has an absorption peak at [31,32], and for the cytosine-guanine bond vibration band it is at [31]. It is observed that these two peaks showed significant spectral shifts as the NaOH concentration increased or, equivalently, the relative denaturation increased in the solution precursors. Both the cytosine vibration peak and cytosine-guanine bond peak shifted toward a lower wavenumber for higher NaOH concentrations as shown in Fig. 4(a). In Fig. 4(b), we plotted the cytosine vibration peak position as a function of NaOH concentration. It has been reported that denaturation of DNA resulted in the spectral peak shift of the nucleobases’ vibrational modes in the FTIR spectra [33], which is consistent with our measurements in solid DNA films. Note that this consistency in FTIR measurements directly indicates that the denaturation of DNA was immobilized into solid films irreversibly. We measured the FTIR spectra for the same solid freestanding films after varying the temperature from 20°C to 60°C for an hour, yet we did not observe any reversal of the spectral shifts in FTIR spectra, which strongly confirms that the denaturation of DNA was irreversibly maintained in the solid thin film.
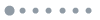
Figure 4.(a) FTIR absorption spectra of solid freestanding DNA films made from DNA aqueous solutions with various NaOH concentrations. (b) Spectral shift of the cytosine vibration peak as a function of NaOH concentration in the DNA precursor solutions.
C. Ellipsometry to Measure Optical Properties
For DNA thin solid films fabricated by spin-coating on Si substrates, the refractive indices and film thickness were measured using a Wollam ellipsometry system, and the results are summarized in Fig. 5 and Table 1. Here we used the isotropic Cauchy model [7,10] because there are no resonant absorption bands in the DNA thin films in the spectral range from 380 to 900 nm. It has been reported that the refractive indices of DNA thin films depend on the film thickness [11] since the surface interaction between the substrate and the film is influenced by the film thickness. In order to focus on the impacts of DNA denaturation on the refractive index, we optimized the spin-coating process to fabricate films with nearly the same thickness of . We found that the viscosity of the NaOH-DNA precursor solution decreased as the NaOH concentration increased or, equivalently, the level of denaturation increased. The thin film process was optimized for NaOH-DNA solution precursors with various viscosities by varying the spinning speed in the range from 750 to 800 r/min, which compensates for the viscosity difference and provides nearly the same film thickness.
NaOH in Precursor Solution (mM) | Thin Film Thickness (nm) |
0 | 43.8±0.6 |
2.5 | 38.6±2.3 |
5.0 | 42.8±3.4 |
7.5 | 39.6±1.6 |
Table 1. Average Thickness of DNA Thin Solid Films Made from Precursor Solutions with Various NaOH Concentrations
NaOH Concentration in Precursor Solution (mM) | dn/dT (1st Cycle, 10−4 °C−1) | dn/dT (2nd Cycle, 10−4 °C−1) |
0 | −4.06 | −3.86 |
2.5 | −4.66 | −4.37 |
5.0 | −5.06 | −4.76 |
7.5 | −5.51 | −5.24 |
Table 2. Thermo-Optic Coefficient of DNA Thin Solid Film at a Wavelength of 633 nm for Various NaOH Concentrations in the Precursor Solutions
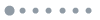
Figure 5.(a) The refractive indices of DNA thin solid film in the spectral range from 380 to 900 nm for various NaOH concentrations in precursor solutions. (b) The refractive indices of DNA thin solid film as a function of NaOH concentration in the precursor solutions.
First we confirmed our measurements for pristine DNA films, at , were consistent with prior reports [7,24,34], which ensures the validity of our experiments. In Fig. 5(a), the refractive index as a function of the wavelength is plotted for DNA films with various NaOH concentrations in the precursor solutions. We observed a consistent decrease in the refractive index of DNA films with increasing NaOH concentrations in the precursor solutions. Note that the refractive index change is not due to the film thickness variation, since all the films were fabricated at a nearly same thickness, and therefore it is due to the DNA denaturation. The refractive index decrease in denaturated DNA thin solid film is quite predictable, since DNA denaturation breaks the nucleobase bonds, and subsequently, the net density decreases [35] in the solid thin films. The refractive index of a solid medium is directly proportional to its density [36], and therefore, DNA denaturation could result in a density decrease and subsequently a refractive index decrease. In Fig. 5(a), a downward shift of the nearly whole optical dispersion curve in the spectral range of 380–900 nm was observed as the NaOH concentration increased from 0 to 2.5, 5.0, and 7.5 mM in the precursor solutions. We plotted the refractive index change as a function of NaOH concentration in Fig. 5(b) showing excellent linear dependence in a wide spectral range covering both the visible and IR, which was not possible in DNA-vitamin B2 thin films due to the intrinsic absorption of vitamin B2 in the visible range. The refractive index difference between the pristine DNA film and the film with 7.5 mM NaOH concentration was as large as in the whole spectral range, and this difference is sufficiently large to make all-DNA waveguide structures, such as a pristine DNA core and denatured DNA cladding.
When the NaOH concentration further increased beyond 7.5 mM, we found the viscosity of the precursor solution sharply decreased, which made it very difficult to fabricate thin films with the same thickness of using the spin-coating system. We did measure the refractive index of DNA thin film with a higher NaOH concentration and confirmed that it continued to decrease but the film thickness was much thinner. We confirmed the irreversible maintenance of DNA denaturation in the solid thin film by experimental FTIR measurements in Fig. 4, which is consistent with the refractive index decreases in Fig. 5 due to the DNA denaturation and subsequent density decrease. However, NaOH embedded in the DNA solid thin films could contribute to the refractive index changes due to its inherent refractive index [37,38]. The actual amount of NaOH in the fabricated thin solid film was not identified, but the concentration of NaOH in the solid film would not exceed that of the precursor solutions, which is in the order of .
D. Investigation of Thermo-Optic Coefficients
Variation of the refractive index with the external temperature, the thermo-optic effect, is a very fundamental mechanism to add novel functionalities in photonic devices providing spectral tuning, switching, and sensing applications. Implementing a temperature-controlled unit inside a Wollam ellipsometry system, we measured the changes in the refractive index and the thickness of DNA films made from NaOH-DNA precursor solutions for various NaOH concentrations. The results are summarized in Fig. 6 in the temperature range from 40°C to 90°C. The heating/cooling rate was about , which was controlled by a Peltier thermoelectric device. We observed a linear correlation between the decrease of the refractive index and the temperature increase, which is consistent with prior reports on a negative thermo-optic coefficient, dn/dT, of DNA thin films [9–11]. We also observed a monotonic decrease of the film thickness as the temperature increased, and after the first cycle, the film thickness slightly decreased due to additional drying of the DNA film, which is also consistent with prior reports [10,11]. We repeated measurements in successive temperature cycles and observed no further significant changes in the thermo-optic coefficients and the film thickness within experimental errors after the second cycle. We summarized the dn/dT measurements of the DNA thin films at for various NaOH concentrations in the precursor solutions in Table 2. The difference in between the first cycle and the second cycle was due to water evaporation within the DNA thin film in the first cycle, which is consistent with prior DNA-based films [11]. In repeated temperature cycling experiments, we did not observe any hysteresis in the refractive index and thickness, which indicated that the DNA films were thermally stable and the melting temperature of the film would be above 90°C. We observed that the magnitude of dn/dT of our denatured DNA thin films was significantly higher than that of DNA-CTMA (cetyltrimethylammonium) thin film, which has recently been measured to be dn/dT [9] for a film thickness of 60 nm. The addition of NaOH and the subsequent increase in the level of DNA denaturation especially showed a good linear correlation with the magnitude of the thermo-optic coefficient, which could be attributed to both the random coil structure of ss-DNA and NaOH ingredients in the DNA thin films. Note that the magnitude of dn/dT of these denaturated DNA thin films is also larger than those of conventional thermo-optic polymers [39], which can provide a definite advantage in various device applications.
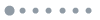
Figure 6.Thermally induced changes in the refractive index and the film thickness of DNA thin solid film with various NaOH concentrations in precursor solutions. (a) Refractive index at as a function of temperature in the first temperature cycle; (b) refractive index at as a function of temperature in the second cycle. (c) Film thickness as a function of temperature in the first cycle; (d) film thickness as a function of temperature in the second cycle.
4. CONCLUSION
We have successfully developed a new method to precisely control the refractive index and thermo-optic coefficient of DNA thin solid film by adopting a denaturation process in the aqueous solution precursor to unwind double-stranded DNAs (ds-DNAs) into single-stranded DNAs (ss-DNAs). These ss-DNAs were irreversibly immobilized in thin solid films by an optimal spin-coating process to systematically alter the physical properties of the film. We found that the denaturation activated by adding NaOH in the solution precursor effectively lowered the refractive index, with in the visible-near-IR region, which is sufficiently high enough to make an all-DNA optical waveguide structure with a ds-DNA core and ss-DNA cladding. We also found that the thermo-optic coefficients dn/dT of DNA thin solid films were systematically controlled from to in the temperature range from 40°C to 90°C by the denaturation process, which can find various applications in biocompatible optical sensing and switching. We experimentally confirmed a high potential to make all-DNA photonic devices by using unique refractive index control of DNA film by the denaturation process.
References
[1] J. D. Watson, F. H. Crick. A structure for deoxyribose nucleic acid. Nature, 171, 737-738(1953).
[2] B. Singh, N. S. Sariciftci, J. G. Grote, F. K. Hopkins. Bio-organic-semiconductor-field-effect-transistor based on deoxyribonucleic acid gate dielectric. J. Appl. Phys., 100, 024514(2006).
[3] J. A. Hagen, W. Li, A. Steckl, J. Grote. Enhanced emission efficiency in organic light-emitting diodes using deoxyribonucleic acid complex as an electron blocking layer. Appl. Phys. Lett., 88, 171109(2006).
[4] A. Steckl, H. Spaeth, H. You, E. Gomez, J. Grote. DNA as an optical material. Opt. Photon. News, 22, 34-39(2011).
[5] Y. Kawabe, L. Wang, S. Horinouchi, N. Ogata. Amplified spontaneous emission from fluorescent dye doped DNA-surfactant complex films. Adv. Mater., 12, 1281-1283(2000).
[6] E. M. Heckman, R. S. Aga, A. T. Rossbach, B. A. Telek, C. M. Bartsch, J. G. Grote. DNA biopolymer conductive cladding for polymer electro-optic waveguide modulators. Appl. Phys. Lett., 98, 103304(2011).
[7] R. Khazaeinezhad, S. H. Kassani, B. Paulson, H. Jeong, J. Gwak, F. Rotermund, D.-I. Yeom, K. Oh. Ultrafast nonlinear optical properties of thin-solid DNA film and their application as a saturable absorber in femtosecond mode-locked fiber laser. Sci. Rep., 7, 41480(2017).
[8] A. Kulkarni, B. Kim, S. R. Dugasani, P. Joshirao, J. A. Kim, C. Vyas, V. Manchanda, T. Kim, S. H. Park. A novel nanometric DNA thin film as a sensor for alpha radiation. Sci. Rep., 3, 2062(2013).
[9] S. Hong, W. Jung, T. Nazari, S. Song, T. Kim, C. Quan, K. Oh. Thermo-optic characteristic of DNA thin solid film and its application as a biocompatible optical fiber temperature sensor. Opt. Lett., 42, 1943-1945(2017).
[10] W. Jung, H. Jun, S. Hong, B. Paulson, Y. S. Nam, K. Oh. Cationic lipid binding control in DNA based biopolymer and its impacts on optical and thermo-optic properties of thin solid films. Opt. Mater. Express, 7, 3796-3808(2017).
[11] E. Hebda, M. Jancia, F. Kajzar, J. Niziol, J. Pielichowski, I. Rau, A. Tane. Optical properties of thin films of DNA-CTMA and DNA-CTMA doped with Nile blue. Mol. Cryst. Liq. Cryst., 556, 309-316(2012).
[12] B. Paulson, I. Shin, H. Jeong, B. Kong, R. Khazaeinezhad, S. R. Dugasani, W. Jung, B. Joo, H.-Y. Lee, S. Park. Optical dispersion control in surfactant-free DNA thin films by vitamin B2 doping. Sci. Rep., 8, 9358(2018).
[13] J. Marmur, P. Doty. Determination of the base composition of deoxyribonucleic acid from its thermal denaturation temperature. J. Mol. Biol., 5, 109-118(1962).
[14] J. Marmur, P. Ts’o. Denaturation of deoxyribonucleic acid by formamide. Biochim. Biophys. Acta, 51, 32-36(1961).
[15] M.-S. Hung, Y.-T. Huang. Laser-induced heating for cell release and cellular DNA denaturation in a microfluidics. BioChip J., 7, 319-324(2013).
[16] J. G. Wetmur, N. Davidson. Kinetics of renaturation of DNA. J. Mol. Biol., 31, 349-370(1968).
[17] H. R. Massie, B. H. Zimm. Kinetics of denaturation of DNA. Biopolymers, 7, 475-493(1969).
[18] M. Ageno, E. Dore, C. Frontali. The alkaline denaturation of DNA. Biophys. J., 9, 1281-1311(1969).
[19] P. Ehrlich, P. Doty. The alkaline denaturation of deoxyribose nucleic acid. J. Am. Chem. Soc., 80, 4251-4255(1958).
[20] F. W. Studier. Sedimentation studies of the size and shape of DNA. J. Mol. Biol., 11, 373-390(1965).
[21] X. Wang, H. J. Lim, A. Son. Characterization of denaturation and renaturation of DNA for DNA hybridization. Environ. Health Toxicol., 29, e2014007(2014).
[22] C. L. Schildkraut, J. Marmur, P. Doty. The formation of hybrid DNA molecules and their use in studies of DNA homologies. J. Mol. Biol., 3, 595-617(1961).
[23] P. Doty, J. Marmur, J. Eigner, C. Schildkraut. Strand separation and specific recombination in deoxyribonucleic acids: physical chemical studies. Proc. Natl. Acad. Sci. USA, 46, 461-476(1960).
[24] A. Samoc, A. Miniewicz, M. Samoc, J. G. Grote. Refractive index anisotropy and optical dispersion in films of deoxyribonucleic acid. J. Appl. Polym. Sci., 105, 236-245(2007).
[25] J. Nizioł, K. Makyła-Juzak, M. M. Marzec, R. Ekiert, M. Marzec, E. Gondek. Thermal stability of the solid DNA as a novel optical material. Opt. Mater., 66, 344-350(2017).
[26] S. Sun, D. Thompson, U. Schmidt, D. Graham, G. J. Leggett. Micro-/nano-patterning of DNA and rapid readout with SERS tags. Chem. Commun., 46, 5292-5294(2010).
[27] T. Masuda, A. Yamaguchi, M. Hayashida, F. Asari-Oi, S. Matsuo, H. Misawa. Visualization of DNA hybridization on gold thin film by utilizing the resistance effect of DNA monolayer. Sens. Actuators B, 105, 556-561(2005).
[28] Y.-W. Kwon, C. H. Lee, D.-H. Choi, J.-I. Jin. Materials science of DNA. J. Mater. Chem., 19, 1353-1380(2009).
[29] P. Y. Vadimovich. UV absorbance of aqueous DNA. Eur. J. Biophys., 3, 19-22(2015).
[30] P. Doty, H. Boedtker, J. Fresco, R. Haselkorn, M. Litt. Secondary structure in ribonucleic acids. Proc. Natl. Acad. Sci. USA, 45, 482-499(1959).
[31] B. Gnapareddy, S. R. Dugasani, T. Ha, B. Paulson, T. Hwang, T. Kim, J. H. Kim, K. Oh, S. H. Park. Chemical and physical characteristics of doxorubicin hydrochloride drug-doped salmon DNA thin films. Sci. Rep., 5, 12722(2015).
[32] G. Tyagi, D. K. Jangir, P. Singh, R. Mehrotra. DNA interaction studies of an anticancer plant alkaloid, vincristine, using Fourier transform infrared spectroscopy. DNA Cell Biol., 29, 693-699(2010).
[33] X. Wang, A. Son. Effects of pretreatment on the denaturation and fragmentation of genomic DNA for DNA hybridization. Environ. Sci. Process. Impacts, 15, 2204-2212(2013).
[34] L. Wang, J. Yoshida, N. Ogata, S. Sasaki, T. Kajiyama. Self-assembled supramolecular films derived from marine deoxyribonucleic acid (DNA)–cationic surfactant complexes: large-scale preparation and optical and thermal properties. Chem. Mater., 13, 1273-1281(2001).
[35] S. Elhadj, G. Singh, R. F. Saraf. Optical properties of an immobilized DNA monolayer from 255 to 700 nm. Langmuir, 20, 5539-5543(2004).
[36] K. Oh, U.-C. Paek. Silica Optical Fiber Technology for Devices and Components: Design, Fabrication, and International Standards(2012).
[37] T. Eggeman. Sodium Hydroxide(2011).
[38] A. L. Olsen, E. R. Washburn. An interpolation table for refractive index-normality relationship for solutions of hydrochloric acid and sodium hydroxide. Trans. Kans. Acad. Sci., 40, 117-126(1937).
[39] Z. Zhang, P. Zhao, P. Lin, F. Sun. Thermo-optic coefficients of polymers for optical waveguide applications. Polymer, 47, 4893-4896(2006).