
- High Power Laser Science and Engineering
- Vol. 9, Issue 4, 04000e64 (2021)
Abstract
1 Introduction
High-power laser systems (from the GW up to the PW range) are used for performing laser–plasma interaction experiments, exploiting the interaction of focused high-intensity laser pulses with solid targets. These experiments aim at studying various fields of physics, such as laser-driven particle acceleration[1], laboratory astrophysics[2–4] and the ion-driven fast ignition approach for inertial confinement fusion[5]. One of the consequences of the laser–target interaction is the generation of pulsed electromagnetic (EM) fields (electromagnetic pulses, EMPs)[6,7]. The mechanisms that drive these EMPs can be different – depending on the interaction regime – and EM radiation with associated different features can be thus emitted at different intensities (up to several MV/m) and frequencies (from the MHz to the THz range)[6,7]. The most well-known EMP emission mechanism is related to the neutralization current that flows through the target holder due to the laser pulse depleting the solid target from electrons[8–12]. This leads to the generation of a fast-oscillating, radiated EM field in the range of radiofrequencies (RFs), that is, from megahertz up to a few gigahertz, that propagates inside the vacuum chamber and also is capable of reaching the space external to the chamber. The amplitude of the electric field can reach the order of megavolts per meter and it represents one main hazard for electronic devices nearby, due to efficient EM coupling in this frequency range. However, intense electric fields can also be generated by the charged particles that are emitted from the irradiated target[7,13–15]: ion wakefields and charge accumulation on surrounding objects can lead to intense quasi-static fields that superimpose on the RF EMP signal. In Ref. [14], Consoli et al. reported measurements performed at the Vulcan PW laser facility, where electrons and protons impinged onto the focusing parabola of the experimental laser setup, which led to transient electric fields in the range of hundreds of kilovolts per meter at a distance of a few meters from the interaction point. In this paper, we present experimental data that exhibit similar characteristics to those reported in Ref. [14], which were obtained during a campaign at the PHELIX laser facility (GSI, Germany)[16]. Our measurements represent a further confirmation that particles emitted from the target are capable of accumulating on objects in the vacuum chamber and therefore generate transient quasi-static electric fields. Moreover, this type of EMP is capable of generating extremely high electric fields at large distances from the target (a distance of over
2 Experimental results
The measurements presented here were obtained during a campaign at the PHELIX laser system (located at the GSI research facility in Germany), which provided – in our specific setup, reported in Figure 1 – pulses with duration of
Figure 1.Experimental setup during the campaign. The focused laser pulse irradiated the solid target, tilted by with respect to the laser axis. Electron and
-ray diagnostics were placed in the laser forward direction, whereas the EMP field probe was placed at about
from the laser axis at a distance of 123 cm from the interaction point. The ions accelerated by the interaction were detected by means of a diamond TOF diagnostic that was elevated above the Teflon (
from the laser axis, 152 cm away from the target). The photograph shows the D-dot probe used in the experiment.
In Figure 2(a) we show the raw signal that was retrieved by the D-dot for shot #32 of the campaign, which had a laser energy of
Sign up for High Power Laser Science and Engineering TOC. Get the latest issue of High Power Laser Science and Engineering delivered right to you!Sign up now
Figure 2.(a) Time domain signal retrieved by the D-dot probe for shot #32. The timescale has been adjusted in order to overlap
The temporal evolution of the electric field needs to be reconstructed from the D-dot signal by numerical time integration[14]:
where
Figure 3.(a) Electric field () as a function of time, reconstructed from the time signal of the D-dot probe placed between the Teflon and the external chamber wall. The
) and the transient component (
) of shot #32, plotted separately. The signals have been obtained from the
signal of panel (a) by applying a low-pass FIR filter (for the transient component) and a high-pass FIR filter (for the RF component).
Figure 4.(a) Schematic sketch of the charge accumulation effect that occurs on the frontal face of the Teflon. The accelerated protons generate a positive quasi-static charge on the Teflon that, in combination with the chamber wall, acts as a capacitor plate, generating the measured field. (b) Typical proton spectrum obtained during the experimental campaign at from the laser axis, that is, behind the D-dot probe. (c) Top: the temporal evolution of
(shot #32) divided into temporal intervals that are associated with the proton populations that were routinely accelerated during the experiment. Below: the TOF signal obtained with the diamond detector placed behind the D-dot. (d) Top: the temporal evolution of
(shot #33) divided into temporal intervals that are associated with the proton populations that were routinely accelerated during the experiment. Below: the TOF signal obtained with a diamond detector placed behind the D-dot.
The presence of accelerated protons (from kiloelectronvolt energy up to multiple megaelectronvolts) and ions, in both laser forward and backward directions, having a broad angular distribution especially for the low-energy part of the spectrum, is compatible with the acceleration regimes that are typical at laser intensities of
The electric field temporal evolution retrieved from shot #33 and reported in Figure 4(d) is qualitatively similar to the previous case: two main rising edges of the electric field are recognizable. The first slope, in the time span indicated with (a) in the top plot of Figure 4(d), up to
In Figure 5 we compare the electric fields of shots #32 and #33 with two additional shots where the component
Figure 5.Comparison between the field, measured during different shots. The field of #31 and #33 (i.e., the shots where the D-dot was rotated) is multiplied by –1 in order to obtain the same field orientation for all shots.
Shot | Laser energy [J] | Target | ||
---|---|---|---|---|
#28 | 23.8 | CHO foam (2 mg/cc, 350 μm) | 31 | 49 |
#31 | 19.1 | CHO foam (2 mg/cc, 350 μm) + Au converter (2 mm) | 21 | 47 |
#32 | 19.3 | CHO foam (2 mg/cc, 350 μm) + Au converter (1 mm) | 22 | 58 |
#33 | 21.8 | CHO foam (2 mg/cc, 350 μm) + Au converter (1 mm) | 19 | 72 |
Table 1. Laser energy, employed target type and EMP electric field characteristics of shots #28, #31, #32 and #33.
3 Numerical simulations
In order to confirm the mechanism that leads to the presence of the dominating transient component of shots #32 and #33, we performed 3D PIC simulations (coupled with a full EM solver) with the commercial code suite CST Particle Studio
Figure 6.Schematic view of the particle-in-cell simulations. The simplified model includes the external chamber wall behind the field probe (the orange circle) and the Teflon wall (having dimensions 30 cm × 30 cm × 10 cm, height × width × thickness). The particle emission point (the red circle) is placed at the left-hand limit of the simulation box. The particles propagate from left to right, that is, in the
In terms of energy, in the regime that we are discussing, multiple electron and ion species are typically accelerated, in both laser forward and backward directions, up to multiple tens of MeV[1]. In this basic model, where the goal was to study the main contribution to the generation of the quasi-static electric field, we simulated different slow ion populations with energies that are compatible with the spectrum reported in Figure 4(b) and only kiloelectronvolt-energy electrons, for both shots. Ionizing radiation was not included since it can hardly be implemented in our type of simulations. Moreover, the ionization of the Teflon or the field probe due to
Figure 7.Comparison between the temporal evolution of the experimental electric field and the simulation results (black line) for shot #32 (a) and shot #33 (b). The timescale of the simulations, similarly as we did for the experimental field, was adjusted in order to superimpose
Shot #32 ( | Shot #33 ( | |
---|---|---|
Electrons | ||
Protons | ||
Table 2. Particle populations implemented in the PIC simulations of shots #32 and #33. The energy distributions have a uniform spread in all cases.
The simulation we ran for shot #33 (for which we changed the sign of the measured field, thus recovering the field inversion due to the
In both shots, protons are accumulated on the frontal face of the Teflon wall, which behaves as the plate collector of a capacitor structure: the field maps that are reported in Figure 8 confirm this phenomenon. We report the spatial distribution of the
Figure 8.Field maps of the component, retrieved from the PIC simulation of shots #32 and #33, at the instants
((b) and (e)) and
4 Details of the experimental and numerical techniques
4.1 D-dot electric field probe measurements and cable calibration
We used a customized version of the AD-80D(R) D-dot field sensor[22] (having a 3-dB-bandwidth cut-off at 5.5 GHz), which we placed between the Teflon and the external metallic wall of the vacuum chamber (see Figure 1). The sensitive components of the probe had no direct line of sight to the interaction point (due to the shielding by the Teflon) in order to avoid direct exposure to particles and ionizing EM radiation emitted from the plasma, which – in the case of conductive probes – may result in measurement artifacts and spurious currents on the sensor[13,39]. With respect to the target, it had a distance of
The field signals of Figure 3(a) exhibit an almost symmetrical change of sign between the two consecutive shots, when the probe was rotated, indicating that the transient growth of the electric field cannot be due to a failure or systematic error of the probe measurement or the numerical field reconstruction.
4.2 TOF proton spectrum measurements using a diamond detector
The TOF measurements were carried out by means of a single crystal chemical vapor deposition diamond detector, having a thickness of
4.3 Particle-in-cell simulations
For the numerical study we used the CST Particle Studio
5 Conclusions
In this work we analyzed the electric field of the EMP signals generated by the laser–solid interaction at the PHELIX laser facility. The temporal evolution of the obtained electric field indicates two main EMP generation mechanisms. The electric field features an RF component that is generated by the neutralization current flowing in the target structure and represents the ‘classical’, most well-known, EMP component[8–12]. However, here we clearly observe that the EMP mechanism that generates the maximum amplitude of the measured electric field is due to the combination of the wakefield of particles (mainly ions) accelerated from the laser-irradiated target and fields due to their accumulation on the frontal face of the Teflon wall, placed in front of the E-field probe.
The accelerated ions carry wakefields, which are sensed by the D-dot probe, before they reach the Teflon, leading to the immediate increase of the longitudinal electric field; they are then accumulated on the Teflon wall, leading to a residual quasi-static field. This object acts as a capacitor structure, where an intense electric field is established. The comparison of this RF component with the quasi-static one shows that the latter is up to a factor of approximately three times more intense than the first (considering the peak-to-peak amplitude of the RF oscillations). This confirms what has already been observed in experiments at Vulcan Petawatt, at different energies and intensities and with a different experimental setup[14], showing that this second source of EMPs can produce remarkable field intensities over a broad range of laser–matter interaction regimes.
This provides useful indications concerning the estimation and the understanding of these fields, which is of high importance for possible mitigation techniques. It is thus very clear that the issue of EMPs needs to be addressed even at large distances from the interaction point: in our case, an electric field of the order of tens of kV/m was measured at a distance of more than
This scheme of charge accumulation, leading to high fields, indicates that the shielding of electronic devices inside the interaction chamber must be designed with accuracy. Dielectric shielding can lead to high fields due to charge accumulation, as we show in this paper, and metallic shielding needs to be equipped with a proper ground connection, in order to ease the discharge of impinging charged particles. On the other hand, these transient quasi-static electric fields of high amplitude, with rise times of the order of tens of nanoseconds and extended spatial distribution, can be potentially implemented for promising applications to several scenarios, such as medical and biological studies[44], studies on material science[45], generation of terahertz radiation[46] and EM compatibility studies[47].
References
[1] A. Macchi, M. Borghesi, M. Passoni. Rev. Mod. Phys., 85, 751(2013).
[2] B. A. Remington, D. Arnet, R. P. Drake, H. Takabe. Science, 284, 1488(1999).
[3] B. Albertazzi, J. Beard, A. Ciardi, T. Vinci, J. Albrecht, J. Billette, T. Burris-Mog, S. N. Chen, D. Da Silva, S. Dittrich, T. Herrmannsdoerfer, B. Hirardin, F. Kroll, M. Nakatsutsumi, S. Nitsche, C. Riconda, L. Romagnani, H.-P. Schlenvoigt, S. Simond, E. Veuillot, T. E. Cowan, O. Portugall, H. Pepin, J. Fuchs. Rev. Sci. Instrum., 84, 043505(2013).
[4] B. Albertazzi, A. Ciardi, M. Nakatsutsumi, T. Vinci, J. Beard, R. Bonito, J. Billette, M. Borghesi, Z. Burkley, S. N. Chen, T. E. Cowan, T. Herrmannsdoerfer, D. P. Higginson, F. Kroll, S. A. Pikuz, K. Naughton, L. Romagnani, C. Riconda, G. Revet, R. Riquier, H.-P. Schlenvoigt, I. Y. Skobelev, A. Y. Faenov, A. Soloviev, M. Huarte-Espinosa, A. Frank, O. Portugall, H. Pepin, J. Fuchs. Science, 346, 325(2014).
[5] S. Atzeni, J. Meyer-ter-Vehn. The Physics of Inertial Fusion: Beam Plasma Interaction, Hydrodynamics, Hot Dense Matter(2009).
[6] F. Consoli, V. T. Tikhonchuck, M. Bardon, P. Bradford, D. C. Carroll, J. Cikhardt, M. Cipriani, R. J. Clarke, T. Cowan, C. N. Danson, R. Da Angelis, M. De Marco, J.-L. Dubois, B. Etchessahar, A. Laso Garcia, D. I. Hillier, A. Honsa, W. Jiang, V. Kmetik, J. Krasa, Y. Li, F. Lubrano, P. McKenna, A. Poyè, I. Prencipe, P. Raczka, R. A. Smith, R. Vrana, N. C. Woosley, E. Zemaityte, Y. Zhang, Z. Zhang, B. Zielbauer, D. Neely. High Power Laser Sci. Eng., 8, e22(2020).
[7] F. Consoli, P. L. Andreoli, M. Cipriani, G. Cristofari, R. De Angelis, G. Di Giorgio, L. Duvillaret, J. Krasa, D. Neely, M. Salvadori, M. Scisciò, R. A. Smith, V. T. Tikhonchuk. , , , , , , , , , , , , and , Philos. Trans. Royal Soc. A , ()., 20200022(2020).
[8] J. L. Dubois, F. Lubrano-Lavederci, D. Raffestin, J. Ribolzi, J. Gazave, A. Compant La Fontaine, E. d'Humières, S. Hulin, P. Nicolai, A. Poyé, V. T. Tinkhonchuk. Phys. Rev. E, 89, 013102(2014).
[9] A. Poyé, J.-L. Dubois, F. Lubrano-Lavaderci, E. D'Humières, M. Bardon, S. Hulin, M. Bailly-Grandvaux, J. Ribolzi, D. Raffestin, J. J. Santos, P. Nicolai, V. Tinkhonchuk. Phys. Rev. E, 92, 043107(2015).
[10] A. Poyé, J.-L. Dubois, F. Lubrano-Lavaderci, E. D'Humieres, M. Bardon, S. Hulin, M. Bailly-Grandvaux, J. Ribolzi, D. Reffestin, J. J. Santos, P. Nicolai, V. Tinkhonchuk. Phys. Rev. E, 92, 059902(2015).
[11] A. Poyé, S. Hulin, M. Bailly-Grandvaux, J.-L. Dubois, J. Ribolzi, D. Raffestin, M. Bardon, F. Lubrano-Lavaderci, E. D'Humières, J. J. Santos, P. Nicolai, V. Tinkhonchuk. Phys. Rev. E, 91, 043106(2015).
[12] A. Poyé, S. Hulin, M. Bailly-Grandvaux, J.-L. Dubois, J. Ribolzi, D. Raffestin, M. Bardon, F. Lubrano-Lavaderci, E. D'Humieres, J. J. Santos, P. Nicolai, V. Tikhonchuk. Phys. Rev. E, 97, 019903(E)(2018).
[13] F. Consoli, R. De Angelis, L. Duvillaret, P. L. Andreoli, M. Cipriani, G. Cristofari, G. Di Giorgio, F. Ingenito, C. Verona. Sci. Rep., 6, 27889(2016).
[14] F. Consoli, R. De Angelis, T. S. Robinson, S. Giltrap, G. S. Hicks, E. J. Ditter, O. C. Ettinger, Z. Najmudin, M. Notley, R. A. Smith. Sci. Rep., 9, 8551(2019).
[15] R. Pompili, M. P. Anania, F. Bisesto, M. Botton, M. Castellano, E. Chiadroni, A. Cianchi, A. Curcio, M. Ferrario, M. Galletti, Z. Henis, M. Petrarca, E. Schleifer, A. Zigler. Sci. Rep., 6, 35000(2016).
[16] V. Bagnoud, B. Aurand, A. Blazevic, S. Borneis, C. Bruske, B. Ecker, U. Eisenbarth, J. Flis, A. Frank, E. Gaul, S. Goette, C. Haefner, T. Hahn, K. Herres, H.-M. Heuck, D. Hochhaus, D. H. H. Hoffmann, D. Javorkova, H.-J. Kluge, T. Kuehl, S. Kunzer, M. Kreutz, T. Merz-Mantwill, P. Neumayer, E. Okels, D. Reemts, O. Rosmej, M. Roth, T. Stoehlker, A. Tauschwitz, B. Zielbauer, D. Zimmer, K. Witte. Appl. Phys. B, 100, 137(2010).
[17] A. Casner, T. Caillaud, S. Darbon, A. Duval, I. Thfouin, J. P. Jadaud, J. P. LeBreton, C. Reverdin, B. Rosse, R. Rosch, N. Blanchot, B. Villette, R. Wrobel, J. L. Miquel. High Energy Density Phys., 17, 2(2015).
[18] B. Le Garrec, S. Sebban, D. Margarone, M. Precek, S. Weber, O. Klimo, G. Korn, B. Rus. Proc. SPIE, 8962(2014).
[19] J. P. Zou, C. Le Blanc, D. N. Papadopoulos, G. Cheriaux, P. Georges, G. Mennerat, F. Druon, L. Lecherbourg, A. Pellegrina, P. Ramirez, F. Giambruno, A. Freneaux, F. Leconte, D. Badarau, J. M. Boudenne, D. Fournet, T. Valloton, J. L. Paillard, J. L. Veray, M. Pina, P. Monot, J. P. Chambaret, P. Martin, F. Mathieu, P. Audebert, F. Amiranoff. High Power Laser Sci. Eng., 3, e2(2015).
[20] C. G. Brown, J. Ayers, B. Felker, W. Ferguson, J. P. Holder, S. R. Nagel, K. W. Piston, N. Simanovskaia, A. L. Throop, M. Chung, T. Hilsabeck. Rev. Sci. Instrum., 83, 10D729(2012).
[21] D. C. Eder, A. Throop, J. Kimbrough, M. L. Stowell, D. A. White, P. Song, N. Back, A. MacPhee, H. Chen, W. DeHope, Y. Ping, B. Maddox, J. Lister, G. Pratt, T. Ma, Y. Tsui, M. Perkins, D. O'Brien, P. Patel. , , , , , , , , , , , , , , , , , , and , “Mitigation of electromagnetic pulse (EMP) effects from short-pulse lasers and fusion neutrons,” Technical Report LLNL-TR-411183 ().(2009).
[23] J. D. Jackson. Classical Electrodynamics(1975).
[24] M. Reiser. Theory and Design of Charged Particle Beams(2008).
[25] O. N. Rosmej, M. Gyrdymov, M. M. Gunther, N. E. Andreev, P. Tavana, P. Neumayer, S. Zaehter, N. Zahn, V. S. Popov, N. G. Borisenko, A. Kantsyrev, A. Skobliakov, V. Panyushkin, A. Bogdanov, F. Consoli, X. F. Shen, A. Pukhov. Plasma Phys. Controll. Fusion, 62, 115024(2020).
[26] M. Salvadori, F. Consoli, C. Verona, M. Cipriani, M. P. Anania, P. L. Andreoli, P. Antici, F. Bisesto, G. Costa, G. Cristofari, R. De Angelis, G. Di Giorgio, M. Ferrario, M. Galletti, D. Giulietti, M. Migliorati, R. Pompili, A. Zigler. Sci. Rep., 11, 3071(2021).
[27] F. Consoli, R. De Angelis, P. L. Andreoli, G. Cristofari, G. Di Giorgio. Phys. Proced., 62, 11(2015).
[28] M. De Marco, M. Pfeifer, E. Krousky, J. Krasa, J. Cikhardt, D. Klir, V. Nassisi. J. Phys. Conf. Ser., 508, 012007(2014).
[29] J. Krasa, M. De Marco, J. Cikhardt, M. Pfeifer, A. Velyhan, D. Klir, K. Rezac, J. Limpouch, E. Krousky, J. Dostal, J. Ullschmied, R. Dudzak. Plasma Phys. Control. Fusion, 59, 065007(2017).
[30] M. J. Mead, D. Neely, J. Gauoin, R. Heathcote, P. Patel. Rev. Sci. Instrum., 75, 4225(2004).
[31] T. Ceccotti, A. Levy, H. Popescu, F. Reau, P. D'Oliveira, P. Monot, J. P. Geindre, E. Lefebvre, P. Martin. Phys. Rev. Lett., 99, 185002(2007).
[32] J. Fuchs, Y. Sentoku, E. d’Humières, T. E. Cowan, J. Cobble, P. Audebert, A. Kemp, A. Nikroo, P. Antici, E. Brambrink, A. Blazevic, E. M. Campbell, J. C. Fernández, J.-C. Gauthier, M. Geissel, M. Hegelich, S. Karsch, H. Popescu, N. Renard-LeGalloudec, M. Roth, J. Schreiber, R. Stephens, H. Pépin. Phys. Plasmas, 14, 053105(2007).
[33] J. Fuchs, Y. Sentoku, S. Karsch, J. Cobble, P. Audebert, A. Kemp, A. Nikroo, P. Antici, E. Brambrink, A. Blazevic, E. M. Campbell, J. C. Fernadez, J.-C. Gauthier, M. Geissel, M. Hegelch, H. Pepin, H. Popescu, N. Renard-LeGalloudec, M. Roth, J. Schreiber, R. Stephens, T. E. Cowan. Phys. Rev. Lett., 94, 045004(2005).
[34] A. Mancic, J. Robiche, P. Antici, P. Audebert, C. Blancard, P. Combis, F. Dorchies, G. Faussurier, S. Fourmaux, M. Harmand, R. Kodama, L. Lancia, S. Mazevet, M. Nakatsutsumi, O. Peyrusse, V. Recoules, P. Renaudin, R. Shepherd, J. Fuchs. High Energy Density Phys., 6, 21(2010).
[35] K. Zeil, S. D. Kraft, S. Bock, M. Bussmann, T. E. Cowan, T. Kluge, J. Metzkes, T. Richter, R. Sauerbrey, U. Schramm. New J. Phys., 12, 045015(2010).
[37] D. Margarone, I. J. Kim, J. Psikal, J. Kaufman, T. Mocek, I. W. Choi, L. Stolcova, J. Proska, A. Choukourov, I. Melnichuk, O. Klimo, J. Limpouch, J. H. Sung, S. K. Lee, G. Korn, T. M. Jeong. Phys. Rev. Spec. Top. Accel. Beams, 18, 071304(2015).
[38] M. A. Furman, M. T. F. Pivi. Phys. Rev. ST Accel. Beams, 5, 124404(2002).
[39] T. S. Robinson, F. Consoli, S. Giltrap, S. Eardley, G. S. Hicks, E. J. DItter, O. Ettlinger, N. H. Stuart, M. Notley, R. De Angelis, Z. Najmudin, R. A. Smith. Sci. Rep., 7, 983(2017).
[40] C. Verona, M. Marinelli, S. Palomba, G. Verona-Rinati, M. Salvadori, F. Consoli, M. Cipriani, P. Antici, M. Migliorati, F. Bisesto, R. Pompili. J. Instrum., 15, C09066(2020).
[41] M. Salvadori, F. Consoli, C. Verona, M. Cipriani, P. L. Andreoli, G. Cristofari, R. De Angelis, G. Di Giorgio, D. Giulietti, M. P. Anania, F. Bisesto, G. Costa, M. Ferrario, M. Galletti, R. Pompili, A. Zigler, P. Antici, M. Migliorati. J. Instrum., 15, C10002(2020).
[42] R. S. Sussmann. CVD Diamond for Electronic Devices and Sensors(2009).
[43] C. Carstensen, S. Funken, W. Hackbusch, R. H. W. Hoppe, P. Monk. Computational Electromagnetics(2003).
[44] A. G. Pakhomov, D. Miklavcic, M. S. Markov. Advanced Electroporation Techniques in Biology and Medicine(2017).
[45] K. M. Gupta, N. Gupta. Advanced Electrical and Electronics Materials: Processes and Applications(2015).
[46] A. Houard, Y. Liu, B. Prade, V. T. Tikhonchuk, A. Mysyrowicz. Phys. Rev. Lett., 100, 255006(2008).
[47] H. W. Ott. Electromagnetic Compatibility Engineering(2009).
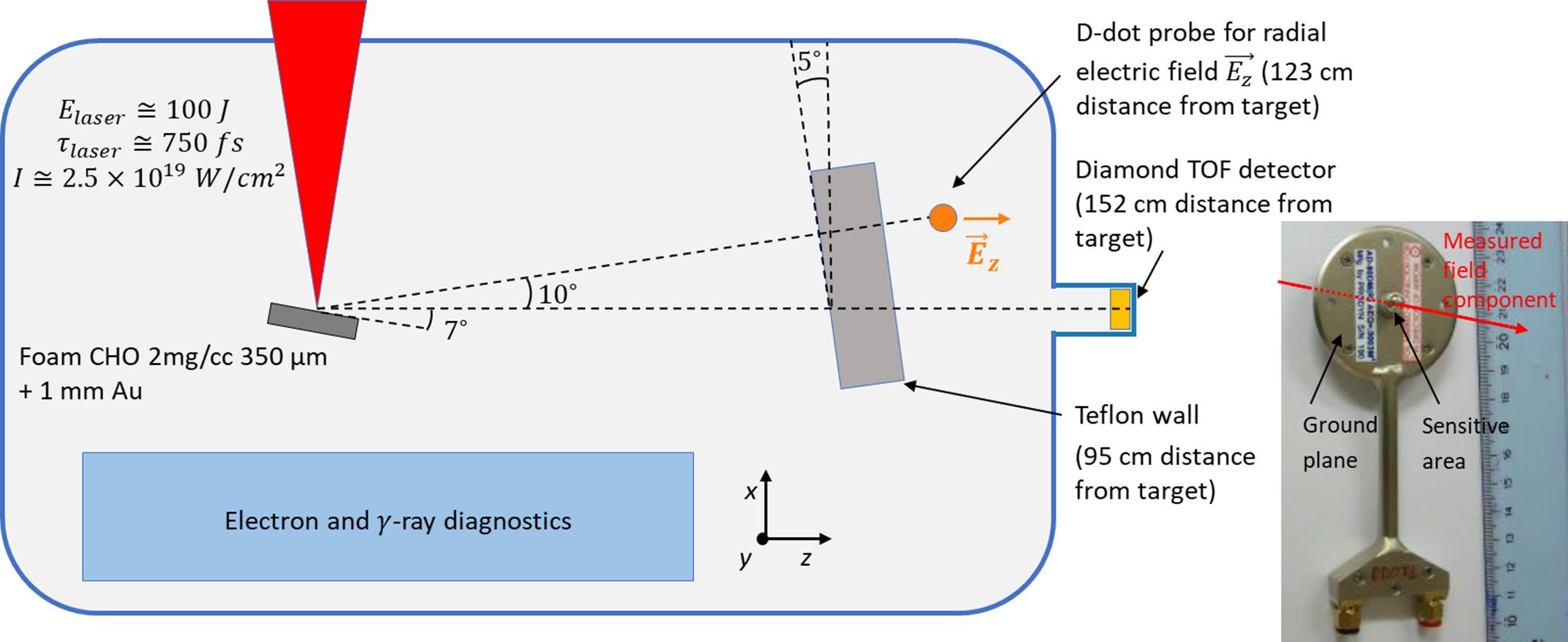
Set citation alerts for the article
Please enter your email address