
- Photonics Research
- Vol. 10, Issue 1, 237 (2022)
Abstract
1. INTRODUCTION
Differential phase contrast (DPC) is a microscopy technique that has recently gained attention for its simple implementation and its ability to provide accurate quantitative phase information regarding transparent samples using incoherent light [1–7]. With the use of asymmetric, incoherent illumination, it is possible to reconstruct the phase profile of weakly scattering samples by inverting approximated linear equations [1]. Several applications have been demonstrated over the years, with particular focus on biological samples [8–12] that often satisfy the requirement of low scattering.
However, it was shown that the phase sensitivity is limited to several tens of mrad [13], which prevents applications where extreme phase sensitivity is required, such as the optical imaging of action potentials, where interferometric techniques still dominate [14–19].
Several steps can be taken to maximize contrast and improve sensitivity. For example, blocking light coming from low angles can increase the contrast by up to a factor of 2 [13]. Another approach is to average multiple frames, but this comes at the cost of speed and makes real-time recording much more data intensive: the sensitivity only improves as the square root of the number of frames.
Sign up for Photonics Research TOC. Get the latest issue of Photonics Research delivered right to you!Sign up now
Finally, sensitivity can be improved by increasing the power of the illumination source, as the phase image is proportional to the total light intensity [1]. On the other hand, the background is also proportional to the illumination power, and for small phase modulations the background component can saturate the detector before significant sensitivity improvement is achieved. While detectors with larger well capacity can be used to extend the dynamic range, these usually have much larger pixels, thus sacrificing either spatial resolution or field of view.
In this paper, we explore a new approach to DPC, based on lock-in amplification to directly measure the amplitude of phase modulation, produced by alternating mirrored illuminations. Using a so-called lock-in camera (heliCam, Heliotis), we demonstrate how it is possible to obtain high-contrast, background-free, full-field DPC images. Other advantages of this technique include a reduced amount of data to be collected, rejection of out of frequency modulations, and optimized use of the dynamic range. Comparative measurements of weak phase transparent samples in standard and lock-in DPC are used to show the improvement given by the proposed technique.
2. THEORY
In DPC, a thin, partially transparent object is usually illuminated with partially coherent light whose angular profile
The background term and the phase transfer function can be calculated starting from the source angular profile and pupil. In particular, if a non-aberrated setup with circular pupil is considered, it is possible to show that
The image formed at the detector is thus the sum of a background term independent of the sample, and a phase term, which is our quantity of interest.
If the source profile is modulated over time, the intensity will also change accordingly. Typically, in DPC the source profile will switch between two or more mirrored profiles. This modulation can be periodic, for example,
The time-dependent image would then be
The quantity of interest is, in this case, the amplitude of the modulated term. In standard DPC this component is isolated from the background by recording a frame for each illumination state, and computing the difference between the two frames, such that
In order to circumvent this problem, it is necessary to use a scheme that directly demodulates the amplitude of
An alternative option is to use a detector with “smart pixels,” which incorporate special electronics to perform more advanced analog operations. One such detector is Heliotis’ heliCam C3, which is a detector based on CMOS technology, and whose pixels contain signal demodulation circuitry [20–23]. The demodulation stage is based on I-Q direct detection, schematically depicted in Fig. 1(c) [22]. The camera outputs the frames
Figure 1.Scheme of operation of lock-in DPC with two illuminations. (a) Simplified DPC microscope scheme during the first half period; the left source
This type of detector can be used to obtain DPC images according to the scheme of Fig. 1(c). Assuming that two sources of illumination are used (for example, from the left and right sides of the optical axis), these can be switched periodically over the period
A. Sensitivity
Sensitivity in DPC can be calculated from the contrast-to-noise ratio (CNR) [13,24,25]: the level of contrast that gives a CNR equal to one is considered to be the minimum amount of modulation that can be detected. If shot noise is the dominating noise source [26,27], it is possible to approximate the CNR as [13,24,28]
Let us now rewrite this expression in terms of source power. The source term
If we assume that the support of
It is indeed possible to increase the CNR by simply increasing the incident power, but in standard DPC the maximum CNR is limited by saturation of the pixels.
The CNR of Eq. (11) holds equally for the lock-in scheme. In this case, the maximum CNR can be limited in three ways: (1) the source has reached its maximum power and cannot be further increased; (2) the modulation is saturating the pixels, in which case the maximum CNR is reached; (3) the background term between the two illuminations is not identical, and their difference
A more detailed derivation of this result is provided in Appendix B. For incoherent sources such as those in our setup, a uniformity level of 1% of the average intensity is presently state-of-the-art [29]. As a consequence, with our current implementation we can expect in practice a maximum improvement in sensitivity by an order of magnitude.
In the lock-in camera by Heliotis, we can set the demodulation period,
In standard DPC, we collect the two images
In the following, we will verify this ratio.
B. Reconstruction
A key step of DPC is the reconstruction of a quantitative phase map of the sample. In order to do this, the DPC image is normalized by the background factor
A simple way to circumvent this issue is to record a single standard DPC image, to use it as reference. The heliCam lock-in camera allows one to record images also as a standard detector. The sources are always driven at their maximum power, which means that the total exposure time in standard DPC has to be much shorter than in lock-in DPC, due to the saturation caused by the strong background. Similar to the ratio of CNR in Eq. (15), the background ratio is equal to the exposure time ratio; thus we can write
This estimated background will be used to reconstruct quantitative phase in lock-in DPC.
In this paper, the method of Tikhonov inversion [1] is used to reconstruct the phase sample from the DPC images. As often observed in the literature [1,2,4], the regularization parameter has been tuned empirically for each experiment, in order to adapt to the various CNR conditions tested [13,30].
3. SETUP
A. Microscope
The microscope DPC setup is shown in Fig. 2. It consists of a classic DPC microscope setup, with the addition of an extra 4f system to relay the pupil located inside the objective barrel. In order to control the NA of the setup, a variable aperture is located at the relayed pupil. After the aperture, a beam splitter separates the light in two paths: on the transmitted path, a tube lens forms an image of the sample at the lock-in camera; on the reflected path, a second 4f system is used to form an image of the pupil plane on a secondary camera. This image represents the illumination profile within the boundaries of the pupil and will be used to calculate the phase transfer function for phase reconstruction [13].
Figure 2.Lock-in DPC microscope setup. The sample is located at the object plane (OP) of the objective (
The heliCam C3 detector features
The microscope’s field of view has a size of
B. Illumination
Several strict requirements have to be met for the illumination of the lock-in DPC setup. In order to ensure the maximum field of view with unsaturated pixels, the background subtraction provided by lock-in only works if the switching illuminations have equal distributions in the object plane. Therefore, it is necessary to build a system that is able to match and align these light distributions to a high degree of accuracy. Moreover, high efficiency in light delivery is necessary, so that enough power is available to take advantage of the lock-in scheme. Finally, the NA should be as close as possible to that of the objective, in order to maximize contrast [4,13].
Considering these conditions, we opted to use two high-power red LEDs, with approximately 120° emission angle. Their emission area is obstructed by electrodes which lead to an inhomogeneous light profile, so it is not possible to directly image them onto the object plane. Among various options to even out the illumination, the use of a hexagonal light pipe was chosen (Edmund Optics, #63-080 N-BK7 Hexagonal Light Pipe). These light pipes can homogenize non-uniform light thanks to total internal reflection, so that the output facet lights up with even intensity. The output facet can then be imaged at the object plane.
The angular distribution of this facet is still symmetric with respect to the optical axis, so it has to be filtered so that the two LEDs provide mirrored distributions that can be both focused at the same position. A convenient solution that combines the filtering and combination of illuminations is the knife-edge mirror. This is made of two mirrors at 90°, and the edge is also reflective (Thorlabs, MRAK25-G01). The edge is placed at the back focal plane of the collimating lens, such that it intersects the optical axis. In this way, the horizontal plane just above the knife-edge mirror will contain only half of the angular distribution of each light pipe output. A focusing lens is then placed at a focal distance from this plane, so that two similar images of the hexagonal facets are created at the object plane.
This solution is very compact, and as a consequence it is possible to use short focal length, high NA lenses, with the benefit of minimal losses and maximum NA. On the other hand, this implementation only features one axis of illumination, which can cause distortions of the phase reconstruction due to missing spatial frequencies in the direction perpendicular to the axis of illumination [1]. This issue could be solved by using custom mirrors with multiple facets, such as pyramidal for two-axis illumination. The I and Q output could then be dedicated to an axis of illumination each, rather than being identical such as in the current implementation. In this paper, we were mostly focused on contrast, and we mitigated distortions for the reconstructions in Section 4.B by making sure that none of the main edges were parallel to the illumination.
4. EXPERIMENTAL RESULTS
The setup described in Fig. 2 was used to characterize the improvements given by the use of lock-in compared to standard DPC.
For each experiment, we collect images of the same sample using both DPC modalities: standard DPC, based on the difference of two images that were separately acquired, and lock-in DPC, based on in-pixel demodulation by the camera.
The lock-in camera features a so-called “intensity mode,” where two standard images at different exposure times are collected. The camera then outputs a high dynamic range image, composed of the non-saturated pixels from the two images. In order to avoid the mixing of these two images, which could introduce extra noise and make the evaluation of CNR incorrect, we maintained the average gray level around one quarter of the 10-bit range, so that only the long exposure time image is used. This means that the exposure time could be increased by a factor of 4, improving the CNR by a factor of 2, according to Eq. (14). Since we are interested in comparing the best result of lock-in DPC with the best result of standard DPC, we will take these factors into account in the following results.
Background images in the absence of a sample are also collected in order to remove unwanted illumination structures, which are particularly intense in lock-in DPC due to the strong amplification. The power of the LEDs is maintained equal during all measurements. This means that the exposure time for standard DPC has to be much shorter due to saturation.
The USAF target samples used in these experiments were fabricated by photolithography of fused silica wafers with varying etching times, in order to obtain different heights and, as a consequence, different phase values. The nominal height was calculated based on the calibrated etching rate, and later verified via atomic force microscopy measurements.
A. Sensitivity
The first set of measurements concerns the improvement of sensitivity that lock-in DPC allows to achieve. A qualitative comparison is shown in Fig. 3, for a USAF target whose phase difference is approximately 19 mrad. In particular, Figs. 3(a) and 3(b) show a region of interest for standard and lock-in DPC, respectively. For the lock-in image, the duration of one cycle is set to be approximately 140 μs, and four cycles are averaged by the camera before transmitting the digitized measurement to the computer. From Eq. (13), the effective exposure time is 280 μs. The sum
Figure 3.Comparison of standard and lock-in DPC images of a 19 mrad USAF target. (a) Region of interest (ROI) of the USAF target measured in standard DPC, after background subtraction and averaging of 280 frames of 1 μs exposure each. (b) ROI of the USAF target measured in lock-in DPC, over a total exposure time of 280 μs, after background subtraction. This image is the sum of the two quadrature images
The standard DPC image is obtained by subtracting the images
The cross section of the USAF target obtained in the two cases is shown in Fig. 3(c), without averaging of the standard DPC case. It is possible to appreciate that, while the shape of the cross section is very similar, the intensity of the positive and negative peaks in lock-in DPC is encoded over approximately 2 orders of magnitude more than those of standard DPC. Moreover, having been able to integrate significantly more light, the relative variation of the gray level between the pixels, caused by noise, is greatly reduced in the lock-in measurement. For an equal amount of collected data, that is
In order to analyze the improvement of contrast in a more quantitative way, we measured the CNR of several USAF samples, with varying phase values, in both DPC techniques. The CNR was calculated as the ratio between the amplitude of the cross section [maximum minus minimum, as seen in Fig. 3(c)], and the standard deviation of a featureless area [31,32]. The ratio of exposure time was again 280. The exposure time chosen for the lock-in DPC was such that some of the peripheral pixels were saturated, and thus we consider this to be the maximum amount of photons that can be integrated before significant distortion. In the standard case, the average gray level reached only one quarter of the full dynamic range, for the reasons explained previously. In order to compare the maximum CNR of both techniques, we corrected the exposure time ratio by a factor of 4, giving 70, and we multiplied all the CNR values of standard DPC by a factor of 2. The results of these measurements are given in Fig. 4(a): it is possible to observe how the CNRs for the lock-in technique are consistently greater than those of standard DPC. In particular, for the smallest sample that we used, at 5 mrad, the CNR of the standard DPC is around 0.82, which means it is already below sensitivity. On the other hand, for the lock-in case, the CNR is around 7.2, which means that the sensitivity limit would be reached around 0.7 mrad.
Figure 4.(a) CNR, measured as the ratio of maximum and minimum peak of the cross section and the standard deviation of a featureless area, is calculated for several samples and plotted against their phase, for both lock-in and standard DPC, in blue and orange, respectively. The CNR of the standard DPC measurements has been multiplied by 2 to take into account the possibility to increase the exposure time without saturating the 10-bit detector. The error bar is obtained over 100 measurements. The green dashed line represents the sensitivity limit at
This is approximately a factor of 8 improvement, which is expected given the exposure time ratio of 70, using Eq. (15). Indeed, Fig. 4(b) shows the experimental ratio of CNR, plotted for all the samples we tested, compared with the theoretical value: the experimental ratio of CNR oscillates around this value. Two of the experimental points require further explanations. The error bar for the first phase value is significantly bigger than the other ones: this is because the amplitude of the cross section in standard DPC suffered from great uncertainty due to its being below sensitivity. This error is then propagated to the ratio of CNR. The last experimental point is further from the theoretical values compared to the other cases. It is possible that the amplitude of the cross section in lock-in DPC is in fact underestimated in this case, as the modulation given by this sample was very close to saturation. Saturation of the lock-in DPC image would explain a CNR value lower than expected.
As we have shown theoretically and experimentally, for an equal amount of collected data, the CNR of lock-in DPC will be greater than that of standard DPC by a factor equal to the square root of the exposure time ratio. On the other hand, it is still possible to reach the same CNR, by averaging enough frames to reach an equivalent total exposure time, as shown in Fig. 4(c). In this experiment, we collected multiple identical frames in standard DPC, and calculated the CNR of an increasing number of averaged frames. The curve of CNR displays the classic
B. Reconstruction
A key feature of DPC is the possibility of reconstructing quantitative phase information about the sample. As explained in Section 2.B, in order to do this, the DPC image has to be normalized by the background
Figure 5.Reconstructed phase of a 50.6 mrad USAF target. The target was placed with an angle of 25° with respect to the direction of illumination, so that none of the edges were parallel to it. As a consequence, no distortion is caused by the missing frequencies. The ROI is rotated here to show straight rectangles. (a) Reconstructed phase from a single 8 μs exposure standard DPC image. (b) Reconstructed phase from 58 averaged standard DPC frames. (c) Reconstructed phase from a single 464 μs lock-in measurement. (d) Comparison of the cross sections indicated in (b) and (c) with the orange and blue lines.
In Fig. 6, we show another interesting feature of lock-in DPC, which is the possibility of modulating contrast by changing the exposure time, without the need to acquire more frames. In particular, Fig. 6(a) shows the phase reconstruction from a single 7 μs exposure standard DPC image, compared with a single lock-in exposure of 120 μs in Fig. 6(b) and 248 μs in Fig. 6(c). The zoomed-in areas show how some low-phase features become more nuanced using lock-in. In both Figs. 6(b) and 6(c), just a single image was collected. The zoomed-in sections are all scaled to the color bar on the left, showing that the normalization procedure allows us to retrieve the same quantitative phase as in standard DPC. As demonstrated previously, it would still be possible to obtain similar results in standard DPC, but this would require acquiring 17 images to reach the contrast of Fig. 6(b) and 35 images to reach that of Fig. 6(c), with the disadvantages that come with a more data intensive measurement, as explained in Section 4.A.
Figure 6.Phase reconstruction of onion epidermal cells. (a) Reconstruction from a single, 7 μs exposure, standard DPC image. (b) Reconstruction from a single, 120 μs exposure, lock-in DPC image. (c) Reconstruction from a single, 248 μs exposure, lock-in DPC image. Second row: zoom from the areas indicated in the orange squares.
5. DISCUSSION AND CONCLUSION
In this paper, we have described a new approach to DPC, which takes advantage of periodic modulation of illumination to automatically extract the DPC information without encoding the background. As a consequence, longer exposure times are possible without saturation of the pixels, which allows increasing the CNR by almost an order of magnitude, in a single shot. In particular, we demonstrated an improvement by a factor of up to 8.3 of CNR for single exposures, which means that around 70 frames are needed in standard DPC to reach the image quality of lock-in DPC.
While similar sensitivities are reachable in standard DPC, it is necessary to average tens of frames, which drastically increases the bit rate of the stream from camera to computer or reduces the duration of recording for cameras with onboard memory. The power of lock-in DPC stands in being able to reach such sensitivity in a single frame, drastically reducing the data- and time-intensiveness of the technique. For example, for a time lapse recording at 1400 frames per second, with a
Example of Bit Rate and Recording Time at 1400 Frames per Second
Standard DPC | Lock-In DPC | |
---|---|---|
Bit rate | 88.2 Gbit/s | 1.26 Gbit/s |
Recording time for 8 GB memory | 0.72 s | 50.79 s |
Recording time for 16 GB memory | 1.45 s | 101.58 s |
This analysis shows that, when resorting to averaging multiple frames to obtain the same sensitivity as in lock-in DPC, the result will still be inferior: with direct computer transfer the frame rate will be lower, and with onboard memory the total recording time will be drastically reduced. Both these choices are undesirable for real-time biological imaging.
Currently, these results are limited by the non-uniformity of the illumination, which could be improved with better illumination setups or by actively matching the sources, for example, using a spatial light modulator. If better illumination is achieved, even longer exposure time can be sustained, further increasing the single-shot sensitivity.
Another limit of this technique is the resolution and field of view given by currently available lock-in cameras. The in-pixel demodulation circuit requires a certain amount of space, giving a pixel size of 40 μm × 40 μm and a total detector size of 300 × 300 pixels. On the other hand, this technical limitation may be overcome in the future.
Moreover, other types of applications can be foreseen for this type of imaging, which can be applied in any situation where weak modulations over strong backgrounds have to be measured. For example, the sample response to illuminations of different polarization or wavelength may be analyzed to map morphological or chemical structures within the sample.
Overall, this approach may enable easier measurement of weak phase details or dynamic phenomena, such as in biological samples, where only interferometric techniques were applied until now [14–16]: for example, Ling
While further improvement of the illumination setup and spatial resolution may be necessary, future applications of this technique will be of interest for several scenarios in biology.
APPENDIX A: LOCK-IN OPERATION FOR DPC
In this section, we provide a more detailed explanation of how the lock-in camera can be used for DPC.
Let us assume that the sample under observation is the simulated phase sample depicted in Fig.
Figure 7.(a) Simulated phase sample; (b) simulated image formed under left-illumination conditions (
The lock-in camera integrates four samples from the input signals, each with a duration of one quarter of the local oscillator period. The key to performing lock-in DPC is to set the light switching period to be equal to the local oscillator period, and to make sure that they are synchronized in phase. In this way, the first two samples (
The local oscillator generates two periodic signals with period
Unlike applications such as optical coherence tomography, in this case the only quantity of interest is the amplitude of the signal. Moreover, if timing of the illumination has been done correctly, I and Q are identical, and from Eq. (
APPENDIX B: CNR IMPROVEMENT LIMIT
As briefly introduced in Section
We define the single-shot CNR improvement as the ratio between the maximum lock-in CNR and the maximum standard CNR, for the same sample. Let us first write the CNR limit for the standard method, using Eq. (
Of course, this is a limit case, since non-uniformity and phase structures may increase the intensity in some pixels at the saturation level. We will express the amplitude of the background non-uniformity as a percentage r of the background
Figure 8.(a) Simulated pixel values along a cross section, for standard DPC image recorded over an exposure time
In the lock-in case, for the same exposure time T, the cross section would look as in Fig.
As a consequence, the maximum CNR improvement is given as
References
[1] L. Tian, L. Waller. Quantitative differential phase contrast imaging in an LED array microscope. Opt. Express, 23, 11394-11403(2015).
[2] Z. F. Phillips, M. Chen, L. Waller. Single-shot quantitative phase microscopy with color-multiplexed differential phase contrast (cDPC). PLoS ONE, 12, e0171228(2017).
[3] Y.-H. Chuang, Y.-Z. Lin, S. Vyas, Y.-Y. Huang, J. A. Yeh, Y. Luo. Multi-wavelength quantitative differential phase contrast imaging by radially asymmetric illumination. Opt. Lett., 44, 4542-4545(2019).
[4] Y. Fan, J. Sun, Q. Chen, X. Pan, L. Tian, C. Zuo. Optimal illumination scheme for isotropic quantitative differential phase contrast microscopy. Photon. Res., 7, 890-904(2019).
[5] Y. Fan, J. Sun, Q. Chen, J. Zhang, C. Zuo. Wide-field anti-aliased quantitative differential phase contrast microscopy. Opt. Express, 26, 25129-25146(2018).
[6] L. Tian, J. Wang, L. Waller. 3D differential phase-contrast microscopy with computational illumination using an LED array. Opt. Lett., 39, 1326-1329(2014).
[7] H.-H. Chen, Y.-Z. Lin, Y. Luo. Isotropic differential phase contrast microscopy for quantitative phase bio-imaging. J. Biophotonics, 11, e201700364(2018).
[8] D. Carpentras, T. Laforest, M. Künzi, C. Moser. Effect of backscattering in phase contrast imaging of the retina. Opt. Express, 26, 6785-6795(2018).
[9] T. Laforest, M. Künzi, L. Kowalczuk, D. Carpentras, F. Behar-Cohen, C. Moser. Transscleral optical phase imaging of the human retina. Nat. Photonics, 14, 439-445(2020).
[10] P. Casteleiro Costa, P. Ledwig, A. Bergquist, J. Kurtzberg, F. E. Robles. Noninvasive white blood cell quantification in umbilical cord blood collection bags with quantitative oblique back-illumination microscopy. Transfusion, 60, 588-597(2020).
[11] W. Lee, J. H. Choi, S. Ryu, D. Jung, J. Song, J. S. Lee, C. Joo. Color-coded LED microscopy for quantitative phase imaging: implementation and application to sperm motility analysis. Methods, 136, 66-74(2018).
[12] P. Ledwig, F. E. Robles. Epi-mode tomographic quantitative phase imaging in thick scattering samples. Biomed. Opt. Express, 10, 3605-3621(2019).
[13] C. Bonati, T. Laforest, M. Kunzi, C. Moser. Phase sensitivity in differential phase contrast microscopy: limits and strategies to improve it. Opt. Express, 28, 33767-33783(2020).
[14] S. Batabyal, S. Satpathy, L. Bui, Y. T. Kim, S. Mohanty, R. Bachoo, D. P. Dave. Label-free optical detection of action potential in mammalian neurons. Biomed. Opt. Express, 8, 3700-3713(2017).
[15] T. Ling, K. C. Boyle, G. Goetz, P. Zhou, Y. Quan, F. S. Alfonso, T. W. Huang, D. Palanker. Full-field interferometric imaging of propagating action potentials. Light Sci. Appl., 7, 107(2018).
[16] T. Ling, K. C. Boyle, V. Zuckerman, T. Flores, C. Ramakrishnan, K. Deisseroth, D. Palanker. High-speed interferometric imaging reveals dynamics of neuronal deformation during the action potential. Proc. Natl. Acad. Sci. USA, 117, 10278-10285(2020).
[17] S. Oh, C. Fang-Yen, W. Choi, Z. Yaqoob, D. Fu, Y. Park, R. R. Dassari, M. S. Feld. Label-free imaging of membrane potential using membrane electromotility. Biophys. J., 103, 11-18(2012).
[18] G. Goetz, T. Ling, T. Gupta, S. Kang, J. Wang, P. D. Gregory, B. H. Park, D. Palanker. Interferometric mapping of material properties using thermal perturbation. Proc. Natl. Acad. Sci. USA, 115, E2499-E2508(2018).
[19] B. Bhaduri, C. Edwards, H. Pham, R. Zhou, T. H. Nguyen, L. L. Goddard, G. Popescu. Diffraction phase microscopy: principles and applications in materials and life sciences. Adv. Opt. Photonics, 6, 57-119(2014).
[20] S. Beer, P. Seitz. Real-time tomographic imaging without X-rays: a smart pixel array with massively parallel signal processing for real-time optical coherence tomography performing close to the physical limits. PhD Research in Microelectronics and Electronics Proceedings, 335-338(2005).
[21] S. Beer, S. Waldis, P. Seitz. Video-rate optical coherence tomography imaging with smart pixels. Proc. SPIE, 5140, 69-76(2003).
[22] S. Beer, P. Zeller, N. Blanc, F. Lustenberger, P. Seitz. Smart pixels for real-time optical coherence tomography. Proc. SPIE, 5302, 21-32(2004).
[23] B. Buttgen, P. Seitz. Robust optical time-of-flight range imaging based on smart pixel structures. IEEE Trans. Circuits Syst. I, 55, 1512-1525(2008).
[24] F. Timischl. The contrast-to-noise ratio for image quality evaluation in scanning electron microscopy. Scanning, 37, 54-62(2015).
[25] H. Jiang, N. Lu, L. Yao. A high-fidelity haze removal method based on HOT for visible remote sensing images. Remote Sens., 8, 844(2016).
[26] P. Hosseini, R. J. Zhou, Y. H. Kim, C. Peres, A. Diaspro, C. F. Kuang, Z. Yaqoob, P. T. C. So. Pushing phase and amplitude sensitivity limits in interferometric microscopy. Opt. Lett., 41, 1656-1659(2016).
[27] L. Azzari, A. Foi. Gaussian-Cauchy mixture modeling for robust signal-dependent noise estimation. IEEE International Conference on Acoustics, Speech and Signal Processing (ICASSP), 5357-5361(2014).
[28] A. Foi, M. Trimeche, V. Katkovnik, K. Egiazarian. Practical Poissonian-Gaussian noise modeling and fitting for single-image raw-data. IEEE Trans. Image Process., 17, 1737-1754(2008).
[29] F. M. Dickey, M. Zimmermann, D. L. Shealy, N. Lindlein, R. Voelkel, K. J. Weible. Microlens laser beam homogenizer: from theory to application. Proc. SPIE, 6663, 666302(2007).
[30] D. P. O’Leary. Near-optimal parameters for Tikhonov and other regularization methods. SIAM J. Sci. Comput., 23, 1161-1171(2001).
[31] W. Lee, D. Jung, S. Ryu, C. Joo. Single-exposure quantitative phase imaging in color-coded LED microscopy. Opt. Express, 25, 8398-8411(2017).
[32] H. Lu, J. Chung, X. Ou, C. Yang. Quantitative phase imaging and complex field reconstruction by pupil modulation differential phase contrast. Opt. Express, 24, 25345-25361(2016).
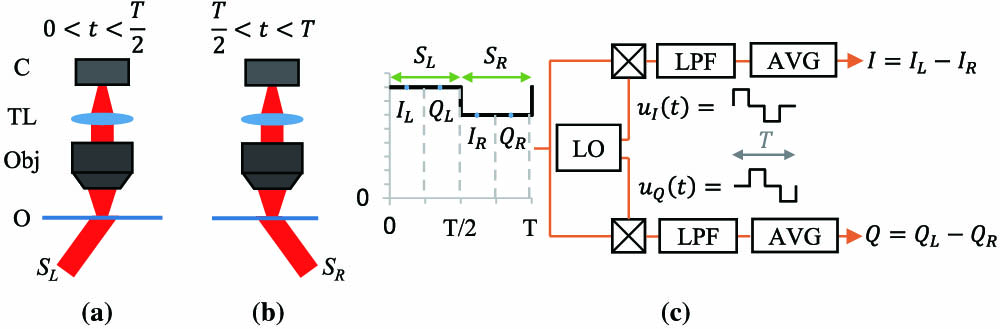
Set citation alerts for the article
Please enter your email address