Xiang You1、2、3、†, Ming-Yang Zheng4, Si Chen2、3, Run-Ze Liu2、3, Jian Qin2、3, Mo-Chi Xu2、3, Zheng-Xuan Ge2、3, Tung-Hsun Chung2、3, Yu-Kun Qiao2、3, Yang-Fan Jiang4, Han-Sen Zhong2、3, Ming-Cheng Chen2、3, Hui Wang2、3, Yu-Ming He2、3, Xiu-Ping Xie4, Hao Li5, Li-Xing You5, Christian Schneider6、7, Juan Yin2、3, Teng-Yun Chen2、3, Mohamed Benyoucef8, Yong-Heng Huo2、3, Sven Höfling6, Qiang Zhang2、3、4, Chao-Yang Lu2、3、9、*, and Jian-Wei Pan2、3、*
Author Affiliations
1University of Science and Technology of China, School of Cyberspace Security, Hefei, China2University of Science and Technology of China, Hefei National Laboratory for Physical Sciences at Microscale, Department of Modern Physics, Hefei, China3University of Science and Technology of China, CAS Centre for Excellence in Quantum Information and Quantum Physics, Shanghai, China4Jinan Institute of Quantum Technology, Jinan, China5Chinese Academy of Sciences, Shanghai Institute of Microsystem and Information Technology (SIMIT), State Key Laboratory of Functional Materials for Informatics, Shanghai, China6Universitat Würzburg, Technische Physik, Physikalisches Instität and Wilhelm Conrad Röntgen-Center for Complex Material Systems, Würzburg, Germany7University of Oldenburg, Institute of Physics, Oldenburg, Germany8University of Kassel, Institute of Nanostructure Technologies and Analytics, CINSaT, Kassel, Germany9NYU-ECNU Institute of Physics at NYU Shanghai, Shanghai, Chinashow less
DOI: 10.1117/1.AP.4.6.066003
Cite this Article
Set citation alerts
Xiang You, Ming-Yang Zheng, Si Chen, Run-Ze Liu, Jian Qin, Mo-Chi Xu, Zheng-Xuan Ge, Tung-Hsun Chung, Yu-Kun Qiao, Yang-Fan Jiang, Han-Sen Zhong, Ming-Cheng Chen, Hui Wang, Yu-Ming He, Xiu-Ping Xie, Hao Li, Li-Xing You, Christian Schneider, Juan Yin, Teng-Yun Chen, Mohamed Benyoucef, Yong-Heng Huo, Sven Höfling, Qiang Zhang, Chao-Yang Lu, Jian-Wei Pan. Quantum interference with independent single-photon sources over 300 km fiber[J]. Advanced Photonics, 2022, 4(6): 066003
Copy Citation Text
show less
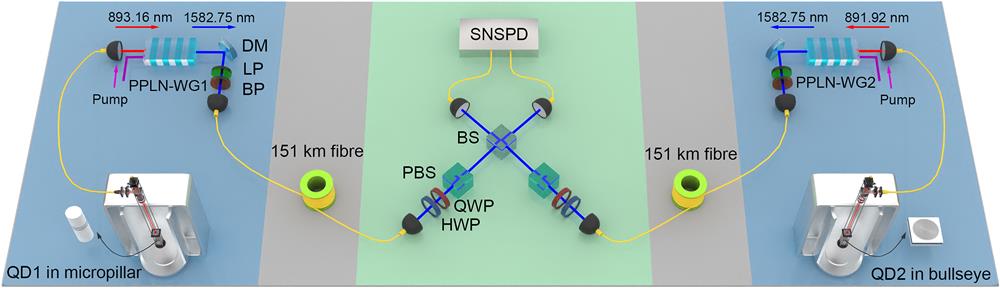
Fig. 1. Experimental configuration of quantum interference between two independent solid-state QD single-photon sources separated by 302 km fiber. Both QDs are embedded in microcavities, with QD1 in a micropillar and QD2 in a bullseye cavity. Under resonant -pulse excitation (not shown), the single photons are emitted from QD1 (QD2), collected by a confocal setup, and then sent into QFC1 (QFC2), which consists of PPLN-WG, pump lasers (not shown, with different wavelengths), and filters (DM, dichromatic mirror; LP, long pass; BP, bandpass). The wavelength of single photons from QD1 (QD2) is converted from near-infrared to 1582.75 nm in QFC1 (QFC2) by adjusting the wavelengths of the pump lasers. The downconverted photons both transmit through 151 km optical fiber and impinge upon a 50:50 beam-splitter (BS) via HOM interference. Arrivals of single photons after interference are detected by two superconducting nanowire single-photon detectors (SNSPDs) and then analyzed using a time-to-digital converter (not shown). The emissions of single photons from QD1 and QD2 are temporally synchronized by pumping with the same laser. The combination of an HWP, a QWP, and a polarization BS makes sure the two single photons will have the same polarization during interference. All fibers are single mode to transform photons into the fundamental transverse Gaussian mode for good spatial-mode overlap.
Fig. 2. Characterization of single photons emitted from QD1 and QD2, respectively. (a) Single photon purity, HBT measurements give and . (b) Indistinguishability, HOM measurements give calculated indistinguishability of 91.9(1)% for QD1 and 83.9(3)% for QD2 after correction. The red (black) data are normalized coincidence counts for two polarizing parallel (orthogonal) photons. (c) Coherence time, measurements are carried out using a Mach–Zehnder interferometer both before QFC1 (QFC2) and after QFC1 (QFC2). By fitting the fringe contrast as temporal delay, we get extracted coherence time of 126(1) [105(2)] ps and 123(3) [103(2)] ps at different positions for QD1 (QD2). The insets show the corresponding single-photon radiative lifetimes for QD1 and QD2, which are calculated by fitting the one-sided exponential decay.
Fig. 3. Characterization of the QFC setup. (a) Fine-tuning of the wavelength of downconverted photons as a function of the position of pump laser’s PZT actuator. The tuning resolution is , corresponding to in the frequency domain. The conversion efficiency is stable in the whole fine-tuning range. (b) Conversion efficiency and (c) signal-to-noise ratio as a function of pump power. The maximum end-to-end efficiency is 48% (52%) at 271 mW (461 mW) for QFC1 (QFC2). The corresponding signal-to-noise values at maximum efficiencies are 29.8 and 28.5 dB, respectively.
Fig. 4. Quantum interference between two solid-state QD single photon sources. (a)–(d) Measurements of coincidence counts between two downconverted photons separated by total fiber lengths of 24 m, 101 km, 201 km, and 302 km, respectively (the 24 m is from the photon collection system before QFC). Red triangles and black dots are the two-photon coincidence counts under the same frequency and 38-GHz detuned, respectively. The integration time for the data set of (a)–(d) is 5 s, 24 s, 9 min, and 2.5 h, respectively. (e) Experimental raw visibilities and theoretical visibility (red line) as a function of fiber length. Both are well above the classical limit of 50%. (f) Dependence of raw visibility on coincidence time window with experimental data extracted from (d). The raw visibility reaches up to at 20 ps.
Fig. 5. Summary of previously reported work and outlook. (a) Summary of quantum interference visibilities between two solid-state QD single-photon sources as a function of distance. (b) Simulations of two-photon coincidence count rate and signal-to-noise ratio as a function of optical fiber length with different system parameters. The solid lines are simulated with parameters of this experiment, including (repetition rate of pulsed excitation laser), (photon system efficiency), (dark counts of SNSPD), and (loss of optical fiber). The dotted lines are simulated with feasibly improved parameters of , , and .