
- Chinese Optics Letters
- Vol. 15, Issue 9, 091402 (2017)
Abstract
The 2–5 μm pulsed lasers with a relative high pulse repetition frequency (PRF) and high pulse energy are of great value for scientific and technical applications, such as laser medical diagnostics, lidar systems, spectroscopy, and mid-infrared countermeasures[
In this Letter, a high energy, high PRF polarized 2.09 μm Ho:YAG laser pumped by a homemade TDFL was first described. Then, the double-resonant ZGP-OPO was presented with this fundamental laser source. The maximum output 2.09 μm laser energy reached about 13.5 mJ at a PRF of 1 kHz, corresponding to continuous wave (CW) power of 16.7 W. The maximum 3–5 μm laser energy reached about 1.25 mJ at a PRF of 3 kHz, corresponding to the peak power of 71.6 kW.
Figure
Sign up for Chinese Optics Letters TOC. Get the latest issue of Chinese Optics Letters delivered right to you!Sign up now
Figure 1.Layout of the TDFL-pumped Ho:YAG oscillator and amplifier system. (
The spectra of the TDFLs were first measured with a high-resolution spectral analyzer (AQ6375, Yokogawa). Figure
Figure 2.(Color online) Typical output spectra of
The main CW output characteristics of the Ho:YAG oscillator were shown in Fig.
Figure 3.(Color online) CW output characteristics of polarized the Ho:YAG oscillator. (a) The pump absorption efficiency of the Ho:YAG crystal, the CW output power, and the transmittance of
The main Q-switched output characteristics of the Ho:YAG oscillator were shown in Fig.
Figure 4.(Color online) Pulsed output characteristics of the polarized Ho:YAG oscillator. (a) Pulse energy and pulse duration versus PRF with pump power of 51.6 W; (b) the pulse profiles at eight different PRFs.
The
Figure 5.(Color online) Output characteristics of the polarized Ho:YAG amplifier. (a) Amplified output CW power versus pump power from
In the experiments above, the Ho:YAG laser performance was of value for the study of the mid-infrared nonlinear process. Here, we apply this fiber-bulk holmium laser system to ZGP-OPO to obtain a 3–5 μm pulsed laser. Figure
Figure 6.Layout of the TDFL-pumped Ho:YAG oscillator and amplifier system. (
The ZGP-OPO was first pumped with the pulsed output laser of the Ho:YAG oscillator. The focal length of lens
Figure 7.(Color online) (a) Comparison of typical pump pulse profile and parametric pulse profile; (b) the beam quality measurement of parametric light.
To further scale the mid-infrared pulse energy and the conversion efficiency, the ZGP-OPO was then pumped with the pulsed output laser of the Ho:YAG amplifier. The focal length of lens
Figure 8.(Color online) (a) Total parametric output average power versus incident pump average power at a PRF of 3 kHz; (b) the beam quality measurements at three typical parametric pulse energies.
In conclusion, the maximum polarized 2.09 μm CW power of 16.7 W and polarized 2.09 μm pulse energy of 13.46 mJ at a PRF of 1 kHz are achieved in a TDFL-pumped Ho:YAG laser. By applying this polarized 2.09 μm pulse laser to ZGP-OPO, the maximum 3–5 μm pulse energy of 1.25 mJ at a PRF of 3 kHz is achieved with a conversion efficiency of 41.73% and a slope efficiency of 53.29%. The peak power reaches about 79.13 kW. The parametric pulse energy and conversion efficiency can be further improved by increasing the pump intensity.
References
[1] J. Wu, Y. Ju, B. Yao, T. Dai, Z. Zhang, X. Duan, Y. Wang. Chin. Opt. Lett., 15, 031402(2017).
[2] F. K. Tittel, R. Lewicki, M. Jahjah, B. Foxworth, Y. F. Ma, L. Dong, R. Griffin, K. Krzempek, P. Stefanski, J. Tarka. Terahertz and Mid Infrared Radiation: Detection of Explosives and CBRN (Using Terahertz), 153(2014).
[3] O. Henderson-Sapir, J. Munch, D. J. Ottaway. Opt. Lett., 39, 493(2014).
[4] E. Lippert, G. Rustad, G. Arisholm, K. Stenersen. Opt. Express, 16, 13878(2008).
[5] R. J. De Young, N. P. Barnes. Appl. Opt., 49, 562(2010).
[6] E. Ji, Q. Liu, Z. Hu, P. Yan, M. Gong. Chin. Opt. Lett., 13, 121402(2015).
[7] P. A. Budni, L. A. Pomeranz, M. L. Lemons, C. A. Miller, J. R. Mosto, E. P. Chicklis. J. Opt. Soc. Am. B, 17, 723(2000).
[8] E. Lippert, S. Nicolas, G. Arisholm, K. Stenersen, G. Rustad. Appl. Opt., 45, 3839(2006).
[9] E. Lippert, H. Fonnum, G. Arisholm, K. Stenersen. Opt. Express, 18, 26475(2010).
[10] A. Hemming, J. Richards, A. Davidson, N. Carmody, N. Simakov, M. Hughes, P. Davies, S. Bennetts, J. Haub. Conference Lasers and Electro-Optics, 1(2013).
[11] H. R. Yang, X. M. Liu. Appl. Phys. Lett., 110, 171106(2017).
[12] L. C. Kong, G. Q. Xie, P. Yuan, L. J. Qian, S. X. Wang, H. H. Yu, H. J. Zhang. Photon. Res., 3, A47(2015).
[13] G. Breitenbach, S. Schiller, J. Mlynek. J. Opt. Soc. Am. B, 12, 2095(1995).
[14] B. Q. Yao, J. H. Yuan, J. Li, T. Y. Dai, X. M. Duan, Y. J. Shen, Z. Cui, Y. B. Pan. Opt. Lett., 40, 348(2015).
[15] S. Brosnan, R. Byer. IEEE J. Quantum Electron., 15, 415(1979).
[16] J. Pearson, U. Ganiel, A. Yariv. IEEE J. Quantum Electron., 8, 433(1972).
[17] R. D. Peterson, K. L. Schepler, J. L. Brown, P. G. Schunemann. J. Opt. Soc. Am. B, 12, 2142(1995).
[18] K. T. Zawilski, S. D. Setzler, P. G. Schunemann, T. M. Pollak. J. Opt. Soc. Am. B, 23, 2310(2006).
[19] A. Hildenbrand, C. Kieleck, A. Tyazhev, G. Marchev, G. Stoppler, M. Eichhorn, P. G. Schunemann, V. L. Panyutin, V. Petrov. Opt. Eng., 53, 122511(2014).
[20] J. Bjorkholm. IEEE J. Quantum Electron., 7, 109(1971).
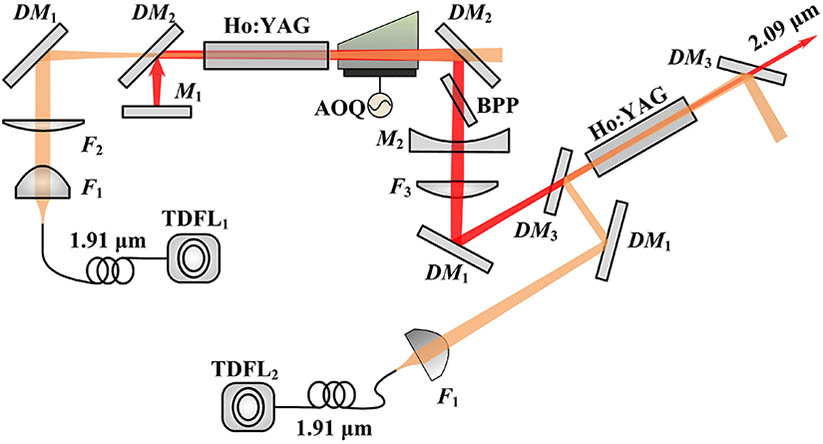
Set citation alerts for the article
Please enter your email address