
- Photonics Research
- Vol. 10, Issue 1, 155 (2022)
Abstract
1. INTRODUCTION
The performance of phosphor-converted white light-emitting diode (LED) technology rises steadily toward its physical limits. Luminous efficacy (LE) has almost doubled in the last 10 years, but the achieved values are still considerably lower than the theoretically calculated maxima [1]. Further improvements are possible by increasing blue chip and package efficiency, as well as the spectral efficiency by developing fluorescent materials with optimal optical characteristics and a high photoluminescence quantum yield (PLQY).
Efficiency, however, is not the only property that determines the quality of a white LED. The ability to reproduce colors faithfully in comparison with an ideal or natural light source is a second, very important characteristic. The International Commission on Illumination (CIE) has defined an internationally agreed method to quantify the ability of a light source to render color [2]. Their most widely used metric (CIE
Quantum dots (QDs) are often presented in the literature as another alternative for the conventional red nitride phosphors. A main advantage of QDs is their very short decay time [8], which offers high power saturation in the range of
Sign up for Photonics Research TOC. Get the latest issue of Photonics Research delivered right to you!Sign up now
A promising cadmium-free alternative is InP-based QDs. The possibility to use such QDs as color converters for white LEDs was already shown in the literature [17,18]. While providing a proof-of-concept, the resulting QD-LEDs had low efficacy and did not reach the
In this paper, we demonstrate that InP/ZnSe QDs with an emission line
2. METHODS
A. Theoretical Benchmark for Color Rendering and Efficacy
The LE is the ratio of the emitted luminous flux to the consumed electrical power. To investigate the maximal
Figure 1.Blackbody spectrum for CCT of 4000 K that is cropped by the blue LED spectrum on the left side, and the red quantum dot spectrum (represented by a Gaussian distribution) on the right side.
The influence of the center wavelength (
B. Experimental Procedures
1. Synthesis of Red-Emitting InP/ZnSe Core/Shell QDs
2. Preparation of White LEDs
We used LUXEON 3535 LED packages from Lumileds (see Fig. 2), without the phosphor in polymer on top of the blue LED chip. These blue LED chips had an emission peak at 450 nm and a wall-plug efficiency of around 70% (
Figure 2.(a) Image of the “empty” LUXEON 3535 LED module. (b) Simulation model of the LED package: 1, LED chip; 2, bottom reflector; 3, inner side of recycling cavity; 4, diffusing bar; 5, package. (c) Reflectance of the LED package and blue chip.
C. Simulation Model of White LED Configuration
Reliable simulations are required to quantify the various loss mechanisms in an LED package and make predictions of potential performance improvements with a more efficient LED chip or QDs with higher quantum yield. Furthermore, simulations enable us to predict the composition of the color-converter resin needed to obtain a specific emission spectrum, thereby avoiding time-consuming trial-and-error experiments.
To be accurate, the simulation model of a white LED package with multiple luminescent materials must include all interactions—absorption, emission, scattering, and re-absorption—of the blue source light and the photoluminescence light, with the different luminescent materials and the LED package. We implemented such simulations in LightTools [29], a commercially available ray-tracing software package.
The LED package and the simulation model, which includes the optical properties of the package and the blue LED chip, are shown in Fig. 2.
As can be seen, the package contained a single
Figure 3.(a) Simulated and measured spectral power distribution of resin samples with different phosphor loadings: Sample 1 (
D. Single-Event Optical Properties of the Luminescent Color Converters
The spectral luminous flux of a phosphor-converted LED package depends strongly on the scattering, absorption, emission, and PLQY of all luminescent materials included. These optical phenomena can be accurately modeled with the commercial ray-tracer LightTools, including multiple scattering and phosphor re-absorption [34]. The underlying model is a Monte Carlo implementation of the fluorescent radiative transfer equation [35]. To solve correctly, this model needs the scattering coefficient
For obtaining accurate single-event properties via reverse modeling, we prepared a series of thin phosphor films with fixed dimensions and different phosphor loadings. The absorbance of these films was measured in a PerkinElmer spectrophotometer. Photoluminescence spectra were obtained by exiting from the films in an integrating sphere [39] by a blue laser. Figure 3(a) shows an example for YAG:Ce phosphor. For the inorganic phosphor powders, the scattering properties were derived via Mie theory, for which the material density and particle size distribution were provided by the manufacturers. We neglected scattering by the QDs, since the scattering cross section is much smaller than the absorption cross section in this case [40].
We start from the listed (if available) material properties and slightly adjust these properties in the simulation model of the phosphor films until we obtain a good match of the measured and simulated spectral radiant flux [Fig. 3(a)]. A detailed discussion of the estimation accuracy of this method falls beyond the scope of this paper, but similar methodologies have also been described in the literature [41].
An overview of the relevant parameters and obtained spectra for all considered materials in this paper are given in Table 1 and Fig. 4, respectively. Wavelengths in parentheses after the material name represent the emission peak wavelength (PWL). Three different red QDs with PWL at 605, 611, and 618 nm were characterized. As shown, all of these QDs have
Scattering/Absorption Coefficient and PLQY of Fluorescent Materials
InP/ZnSe | YAG:Ce | LuAG:Ce | |
---|---|---|---|
0 | 3.5 | 1.95 | |
0.44 | 0.72 | 0.33 | |
PLQY [%] | 75–80 | 98 | 92 |
Figure 4.Emission/absorption spectrum of YAG:Ce (545 nm), LuAG:Ce (516 nm), and red InP/ZnSe quantum dots.
3. RESULTS AND DISCUSSION
A. Theoretical Color Rendering and Efficacy of QD-LEDs
We used a theoretical emission spectrum (see Section 2) to investigate the maximal
The influence of the center wavelength (
Figure 5.(a)
As the center wavelength of the red emission shifts toward longer wavelengths and the emission line broadens, both
When considering the above results, it is important to acknowledge that the actual values for
B. Experimental White Light Spectra of InP-Based QD-LEDs
For this study, we used InP/ZnSe QDs with an emission linewidth of
As a first reference QD-LED, we prepared color-converter resins containing a YAG:Ce phosphor with PWL at 545 nm (YAG:Ce 545) as a broadband phosphor and red InP/ZnSe QDs with a single-event peak emission at 605 nm and a PLQY of 75%. As can be seen in Fig. 6, the resulting white light spectrum of QD-LED1 consists of a combination of the YAG:Ce and the QD emission—the latter giving an additional band centered at 610 nm. The white LED1 has a fairly high efficiency of 143 lm/W because most of the light is emitted near the maximum of the luminous efficiency function. The color rendering, however, is moderate with
Figure 6.LED1, measured spectral power distribution of the white QD-LED with
To overcome these limitations, we replaced YAG:Ce 545 by a LuAG:Ce phosphor with peak emission at 516 nm (QD-LED2). As can be seen in Fig. 7, the use of LuAG:Ce 516 significantly reduces the cyan gap in the QD-LED2 spectrum and limits the red emission tail coming from the YAG:Ce 545 emission in QD-LED1. This results in
Figure 7.LED2, white QD-LED with the cyan region perfectly filled by using the LuAG:Ce phosphor, resulting in
As previously mentioned,
Figure 8.LED4, measured spectral power distribution of the white LED using LuAG:Ce (516 nm), YAG:Ce (545 nm), and red-emitting QDs (611 nm) as luminescent materials. This LED balances color rendering and LE performance.
Figure 9.Spectra of different white LEDs with similar color rendering performance (
C. Luminous Efficacy of Radiation
Since the white light QD-LED4 spectrum meets current color rendering standards, we used that spectrum as a starting point to compare the LER for different white LEDs. The LER of a given spectrum is defined as the ratio of the luminous flux to the radiant flux, a ratio that depends on the position and the width of the QD emission band. The white LEDs that are considered in this comparison all have similar color rendering properties (
In Fig. 9, we represent the different 3000 and 4000 K white LED spectra, normalized to the same total output power, while Table 2 summarizes the corresponding LERs. Considering the 3000 K LEDs first, one sees that the narrower red emission of the two white QD-LEDs results in considerably larger LER as compared to the white LED with a nitride phosphor. More precisely, we find that the LER of the InP-based QD-LED spectrum is 17% larger than the nitride-based LED. Clearly, this is the result of the broad, red emission tail of the nitride phosphor, which extends well beyond the human eye sensitivity curve. On the other hand, the replacement of InP-based QDs by Cd-based QDs only results in a moderate increase of the LER by 4.5%. In line with our theoretical estimates, this limited impact of a more narrow emission line on the LER can also be linked to the slight redshift of the emission line that is needed to preserve good color rendering. In the case of the 4000 K white LEDs, we similarly find that the LER of the nitride-based white LED is 18.2% smaller than that of the InP-based QD-LED. Opposite from our theoretical results, however, we find that the LER of the OSRAM Osconiq LED is smaller than of QD-LED4, even if the former LED uses Cd-based QDs. Looking at the spectrum of the OSRAM Osconiq LED, this is also the result of the relatively broad, red emission peak that is also present in this case. A possible reason for this is the wider than expected emission line of the Cd-based QDs, but also the extension of the emission of the powder phosphor(s) in the far-red region is certainly possible. We, thus, conclude that, while the replacement of broad emitting, nitride-based phosphors by QDs can significantly improve the LER of white LEDs, it is the overall phosphor-QD mixture that determines the final LER gains. In particular, the modest efficacy improvements expected for more narrow QD emission lines can easily be undone by the choice of the green-yellow phosphor in the color converter.
Luminous Efficacy of Radiation of White LEDs with Different Luminescent Materials for the Red Emission
LED Type | LER (lm/W) | Compared to InP QDs |
---|---|---|
274 | −17% | |
340 | ||
325 | ||
285 | −18.2% | |
314 | −8.4% | |
345 |
D. Luminous Efficacy of InP-Based White LEDs
While the LER of the QD-LED4 spectrum amounts to 345 lm/W, the actual QD-LED’s LE was 132 lm/W only, as indicated in Section 3.B. For the LUXEON 3535 white LED with nitride phosphors (
To get more detailed insight into the QD-LED energy power budget, the different loss mechanisms, and the resulting efficacy, we simulated the output spectrum of QD-LED4 using the geometry of the LED package and the properties of the different converter materials as input (see Section 2). Figure 10(a) represents the experimental and simulated spectral radial flux, where the excellent match between both provides a solid basis to quantify the losses. Considering the measured wall-plug efficiency of 70% for the blue LED, the simulated output spectrum yields a total power budget for QD-LED4, as depicted in Fig. 10(b). According to this diagram, we attribute the optical losses—expressed as a fraction of the total power budget—of QD-LED4 to:
Figure 10.(a) Measured spectral power distribution of the demonstrated InP/ZnSe QD-LED4 (
As a result, 38% of the electric power is retrieved as optical power yielding an LE of 132 lm/W.
While Stokes losses or reflection losses are difficult, if not impossible to avoid, a further enhancement of the LE can result from an increased wall-plug efficiency of the blue LED and a higher PLQY of the luminescent materials. Using our simulation model for QD-LED4, we evaluated both aspects of the power budget separately. In Fig. 11(a), the variation of the LE with the blue chip efficiency is shown, when the PLQY of the red QDs equals 80%. As can be expected, the QD-LED efficacy increases linearly with the wall-plug efficiency to reach a maximum of 188.5 lm/W for a 100% efficient electrical to optical power conversion. While this figure remains well below the LER of 345 lm/W, this result also shows that a considerable gain of around 2 lm/W can be made for every percentage increase of the wall-plug efficiency of the blue LED. As compared to such gains, the impact of a further rise of the PLQY of the InP/ZnSe QDs is more limited. In Fig. 11(b), the variation of the LE with the PLQY of the red QDs is shown when the blue chip efficiency equals 70%. The relation between the LE of the QD-LED and the PLQY of the red QDs is clearly non-linear where the gains of increasing the PLQY drop for the highest PLQYs. This non-linear relation is a direct consequence of the multiple re-absorption effects that occur in the white QD-LED. If the PLQY of the red QDs is lower, a higher QD concentration is needed to reach the same color point. This higher QD concentration not only results in more absorption of blue light but also more absorption of the green-yellow light emitted by the YAG:Ce and LuAg:Ce phosphors and also more self-absorption of red light emitted by the QDs themselves. The negative impact of these multiple re-absorption effects on the LE increases considerably when the PLQY of the QDs is lower. As a result, replacing 80% efficient by 100% efficient QDs only increases the LE from 132 to 141 lm/W, whereas using 40% efficient QDs would reduce the LE to 104 lm/W. In order to evaluate the combined impact of both the blue chip and red QD efficiency on the LE, one can simply multiply the linear increase as a consequence of the blue chip efficiency increase, with the non-linear increase as a consequence of the red QD PLQY increase. This would result, for example, in an
Figure 11.Predicted luminous efficacy values of the InP/ZnSe QD-LED with CCT = 4000 K for (a) varying wall-plug efficiency of the blue LED chip and (b) varying quantum yield of the InP/ZnSe quantum dots.
Despite the larger LER of the InP/ZnSe QD-LED, the measured LE was lower than that of the other two considered white LEDs at
4. CONCLUSION
In this study, we show that InP/ZnSe QDs with an FWHM bandwidth of 45 nm and a quantum efficiency of nearly 80% offer a competitive alternative to already well-developed and commercially available Cd-based QDs. Both our theoretical calculations and experimental results show that the efficiency penalty for the wider emission spectrum compared to narrowband Cd-based QDs remains under 5% in all cases. The best result using a Lumileds 3535 package with a blue chip wall-plug efficiency of 70% is an overall LE of 132 lm/W for
In this paper, we considered the optical properties and application of InP/ZnSe QDs for white LEDs with good color rendering and high efficacy. In future work, we will address the reliability of the InP/ZnSe QDs under high flux-densities and elevated temperature, as well as the process ability of these QDs.
Acknowledgment
Acknowledgment. We are grateful to Lumileds for providing us with a large batch of empty 3535 LED packages and relevant information about this specific package. Special thanks also go to Prof. Philippe Smet and Prof. Kevin Smet for some useful suggestions and guidelines.
References
[1] P. Morgan Pattison, M. Hansen, J. Y. Tsao. LED lighting efficacy: status and directions. C. R. Phys., 19, 134-145(2018).
[2] C. I. del Eclairage. Measuring and specifying colour rendering properties of light sources(1995).
[3] K. Houser, M. Mossman, K. Smet, L. Whitehead. Tutorial: color rendering and its applications in lighting. LEUKOS, 12, 7-26(2016).
[4] M. Wright. California tightens color performance of LED based lamps(2016).
[5] M. Wei, K. W. Houser, A. David, M. R. Krames. Perceptual responses to LED illumination with colour rendering indices of 85 and 97. Light. Res. Technol., 47, 810-827(2015).
[6] D. Estrada, K. Shimizu, M. Bohmer, S. Gangwal, T. Diederich, S. Grabowski, G. Tashjian, D. Chamberlin, O. B. Shchekin, J. Bhardwaj. 32-1: on-chip red quantum dots in white LEDs for general illumination. SID Symp. Dig. Tech. Pap., 49, 405-408(2018).
[7] H. F. Sijbom, R. Verstraete, J. J. Joos, D. Poelman, P. F. Smet. K2SiF6:Mn4+ as a red phosphor for displays and warm-white LEDs: a review of properties and perspectives. Opt. Mater. Express, 7, 3332-3365(2017).
[8] X. Fan, T. Wu, B. Liu, R. Zhang, H. C. Kuo, Z. Chen. Recent developments of quantum dot based micro-LED based on non-radiative energy transfer mechanism. Opto-Electron. Adv., 4, 21002201(2021).
[9] A. Tierno, T. Ackemann, C. G. Leburn, C. T. Brown. Saturation of absorption and gain in a quantum dot diode with continuous-wave driving. Appl. Phys. Lett., 97, 231104(2010).
[10] A. P. Alivisatos. Semiconductor clusters, nanocrystals, and quantum dots. Science, 271, 933-937(1996).
[11] S. Abe, J. J. Joos, L. I. Martin, Z. Hens, P. F. Smet. Hybrid remote quantum dot/powder phosphor designs for display backlights. Light Sci. Appl., 6, e16271(2017).
[12] J. Kurtin, B. Mangum, B. Theobald. On-chip quantum dots for high color gamut displays. Dig. Tech. Pap. SID Int. Symp., 48, 447-450(2017).
[13] B. D. Mangum, T. S. Landes, B. R. Theobald, J. N. Kurtin. Exploring the bounds of narrow-band quantum dot downconverted LEDs. Photon. Res., 5, A13-A22(2017).
[14] OSRAM Opto. Quantum dots from OSRAM make LEDs even more efficient.
[15] R. Guide. RoHS initiatives worldwide(2021).
[16] . Compliance FAQs: RoHS(2020).
[17] J. Ziegler, S. Xu, E. Kucur, F. Meister, M. Batentschuk, F. Gindele, T. Nann. Silica-coated InP/ZnS nanocrystals as converter material in white LEDs. Adv. Mater., 20, 4068-4073(2008).
[18] S. Kim, T. Kim, M. Kang, S. K. Kwak, T. W. Yoo, L. S. Park, I. Yang, S. Hwang, J. E. Lee, S. K. Kim, S. W. Kim. Highly luminescent InP/GaP/ZnS nanocrystals and their application to white light-emitting diodes. J. Am. Chem. Soc., 134, 3804-3809(2012).
[19] K. T. Shimizu, M. Böhmer, D. Estrada, S. Gangwal, S. Grabowski, H. Bechtel, E. Kang, K. J. Vampola, D. Chamberlin, O. B. Shchekin, J. Bhardwaj. Toward commercial realization of quantum dot based white light-emitting diodes for general illumination. Photon. Res., 5, A1-A6(2017).
[20] Q. A. Akkerman, G. Rainò, M. V. Kovalenko, L. Manna. Genesis, challenges and opportunities for colloidal lead halide perovskite nanocrystals. Nat. Mater., 17, 394-405(2018).
[21] H. Chen, J. He, S.-T. Wu. Recent advances on quantum-dot-enhanced liquid-crystal displays. IEEE J. Sel. Top. Quantum Electron., 23, 1900611(2017).
[22] G. Harbers, C. Hoelen. LP-2: high performance LCD backlighting using high intensity red, green and blue light emitting diodes. SID Symp. Dig. Tech. Pap., 32, 702-705(2001).
[23] Z. Liu, C. H. Lin, B. R. Hyun, C. W. Sher, Z. Lv, B. Luo, F. Jiang, T. Wu, C. H. Ho, H. C. Kuo, J. H. He. Micro-light-emitting diodes with quantum dots in display technology. Light Sci. Appl., 9, 1(2020).
[24] K. A. Smet. Tutorial: the LuxPy Python toolbox for lighting and color science. LEUKOS, 16, 179-201(2020).
[25] E. Dhaene, J. Billet, E. Bennett, I. Van Driessche, J. De Roo. The trouble with ODE: polymerization during nanocrystal synthesis. Nano Lett., 19, 7411-7417(2019).
[26] M. P. Hendricks, M. P. Campos, G. T. Cleveland, J. L. P. Ilan, J. S. Owen. A tunable library of substituted thiourea precursors to metal sulfide nanocrystals. Science, 348, 1226-1230(2015).
[27] https://formlabs.com/store/clear-resin/. https://formlabs.com/store/clear-resin/
[28] P. Hanselaer, A. Keppens, S. Forment, W. R. Ryckaert, G. Deconinck. A new integrating sphere design for spectral radiant flux determination of light-emitting diodes. Meas. Sci. Technol., 20, 095111(2009).
[30] K. Wang, S. Liu, X. Luo, D. Wu. Freeform Optics for LED Packages and Applications(2017).
[31] J. K. Kim, H. Luo, E. F. Schubert, J. Cho, C. Sone, Y. Park. Strongly enhanced phosphor efficiency in GaInN white light-emitting diodes using remote phosphor configuration and diffuse reflector cup. Jpn. J. Appl. Phys., 44, L649-L651(2005).
[32] C.-C. Sun, T.-X. Lee, S.-H. Ma, Y.-L. Lee, S.-M. Huang. Precise optical modeling for LED lighting verified by cross correlation in the midfield region. Opt. Lett., 31, 2193-2195(2006).
[33] I. Moreno, C.-C. Sun. Modeling the radiation pattern of LEDs. Opt. Express, 16, 1808-1819(2008).
[34] M. W. Zollers, H. Yang, J. H. Melman, S. R. David, G. Wang, X. Xu. Process to measure particulate down-converting phosphors and create well-correlated software models of LED performance. Proc. SPIE, 7954, 795414(2011).
[35] Y. Ma, M. Wang, J. Sun, R. Hu, X. Luo. Phosphor modeling based on fluorescent radiative transfer equation. Opt. Express, 26, 16442-16455(2018).
[36] J. Ryckaert, A. Correia, M. D. Tessier, D. Dupont, Z. Hens, P. Hanselaer, Y. Meuret. Selecting the optimal synthesis parameters of InP/Cd
[37] W. Zhang, D. Dai, X. Chen, X. Guo, J. Fan. Red shift in the photoluminescence of colloidal carbon quantum dots induced by photon reabsorption. Appl. Phys. Lett., 104, 091902(2014).
[38] S. Leyre, Y. Meuret, G. Durinck, J. Hofkens, G. Deconinck, P. Hanselaer. Estimation of the effective phase function of bulk diffusing materials with the inverse adding-doubling method. Appl. Opt., 53, 2117-2125(2014).
[39] S. Leyre, E. Coutino-Gonzalez, J. J. Joos, J. Ryckaert, Y. Meuret, D. Poelman, P. F. Smet, G. Durinck, J. Hofkens, G. Deconinck, P. Hanselaer. Absolute determination of photoluminescence quantum efficiency using an integrating sphere setup. Rev. Sci. Instrum., 85, 123115(2014).
[40] J. Li, Y. Tang, Z. Li, X. Ding, D. Yuan, B. Yu. Study on scattering and absorption properties of quantum-dot-converted elements for light-emitting diodes using finite-difference time-domain method. Materials, 10, 1264(2017).
[41] Z. Liu, S. Liu, K. Wang, X. Luo. Measurement and numerical studies of optical properties of YAG:Ce phosphor for white light-emitting diode packaging. Appl. Opt., 49, 247-257(2010).
[42] I. Moreels, J. C. Martins, Z. Hens. Solution NMR techniques for investigating colloidal nanocrystal ligands: a case study on trioctylphosphine oxide at InP quantum dots. Sens. Actuators B, 126, 283-288(2007).
[43] J. S. Li, Y. Tang, Z. T. Li, K. Cao, C. M. Yan, X. R. Ding. Full spectral optical modeling of quantum-dot-converted elements for light-emitting diodes considering reabsorption and reemission effect. Nanotechnology, 29, 295707(2018).
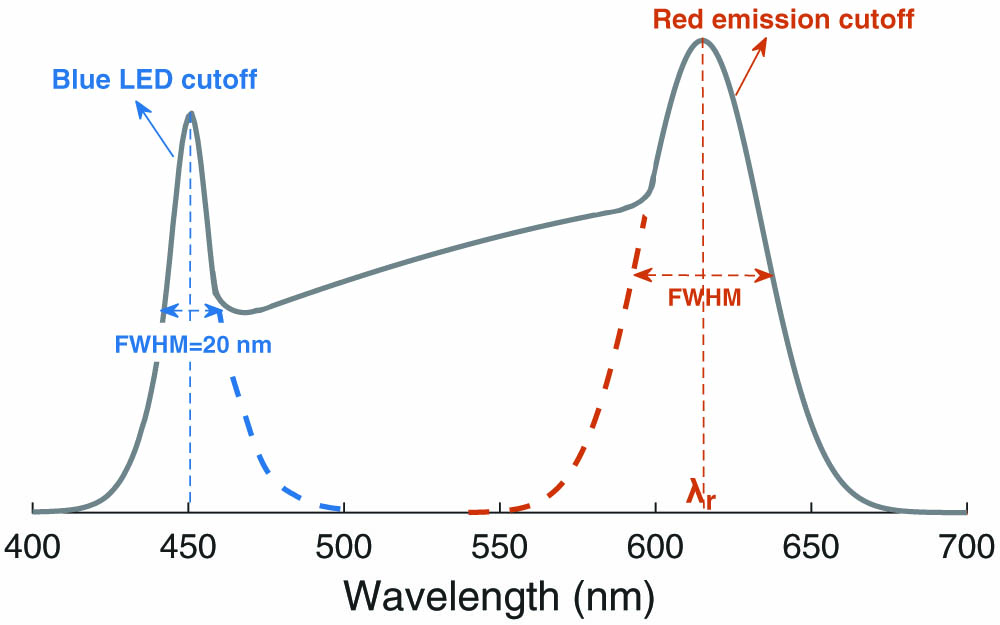
Set citation alerts for the article
Please enter your email address