
- Photonics Research
- Vol. 9, Issue 4, 610 (2021)
Abstract
1. INTRODUCTION
Half a century ago, enthusiasm for optical information processing in parallel architectures promoted the invention of spatial light modulators (SLMs) [1–3]. Such devices enable harnessing light in its amplitude, phase, or polarization both spatially and temporally at will [4,5], by which information could be encoded into an optical wavefront with a certain spatial distribution. Nowadays, the SLMs can steadily find themselves in a broad spectrum of applications in our daily lives and cutting edge researches, such as portable displays, virtual reality, light detection and ranging, and super-resolution imaging [6–9].
Depending on the way that information is written into the devices, the SLMs can be divided into electrically addressed SLMs (EASLMs) and optically addressed SLMs (OASLMs) [10]. Complying with the development of electronic information technology during the past decades, the EA has become the most popular technique in current commercial SLMs. However, for future all-optical information process systems, the EASLMs are not the best choice because information needs to convert back and forth between optical and electronic domains. The OASLMs, on the other hand, allow light modulation directly by light without electronic-optical conversion [11,12]. Furthermore, OASLMs are essential for realizing various all-optical applications that are impossible by EASLMs, including coherent to incoherent image conversion, real time optical correlation, and parallel all-optical computation [13–17]. In principle, OASLMs can be constructed based on nonlinearities of materials, in which the modulation over the read light is fulfilled by spatially selectively changing the properties of the materials via nonlinear optical stimuli [2,18–24]. But, the nonlinearities in natural materials are too weak to support efficient “light-control-by-light” within nanoscale volumes. This makes the devices very cumbersome or requires strong pumping power to accumulate sufficiently large nonlinear modulations, and thus such devices are not suitable for the nano-era.
The recent exciting progress in the dynamic optical metasurface (MS) gives an opportunity to tackle the above obstacles and provides a new framework for the nanoscaled SLM [25,26]. The MS can also boost the optical interactions by concentrating light in nanoscale volumes, which makes it possible for significant control over the light fields under, for example, external mechanical, chemical, and magnetic stimuli [27–31]. Based on the active manipulation of light beams by external electrical fields, the ultracompact EASLMs based on MS have been demonstrated recently [25,26,32].
Sign up for Photonics Research TOC. Get the latest issue of Photonics Research delivered right to you!Sign up now
In this paper, we present a novel OASLM based on MS (MS-OASLM). The working principle of our device relies on the nonlinear control of the polarization of the read light by another write light. The MS-OASLM has a ultra-small thickness of only 400 nm, which is thinner than one tenth of any conventional SLMs [4]. Image projections by our MS-OASLM are demonstrated, which are implemented by transforming the polarization modulation into an intensity replica based on Malus’ law. The resolution achieves 500 line pairs per millimeter (lp/mm), which is more than one order of magnitude better than the typical commercial SLMs (for example Hamamatsu X10468-01, 25 lp/mm). The MS-OASLM would provide a flexible and compact platform for next generation ultrahigh resolution optical displays and all-optical applications including parallel image processing and parallel optical computing.
2. RESULTS
Figure 1.Operation principle of an MS-OASLM. (a) An illustration for the MS-OASLM. Write beams (green arrows) with different intensities cause a spatially heterogeneous photoisomerization (trans state to cis state) of ethyl-red azo molecules and selectively tune optical responses of the MS. This consequently affects readout light polarization in a nonlinear manner, as indicated by the differently rotated red arrow plane in the transmission side. Inset gives an SEM image of the MS unit cell. (b) Transmission spectra of the MS for
Figure 2.Image projection based on an MS-OASLM. (a) Schematic of the MS-OASLM image projection system. An input mask is imaged onto the MS plane using 532 nm green light and is duplicated in form of a spatially inhomogeneous polarization distribution in readout light. Such a polarization replica is transformed into an intensity replica by filtering through a combination of a waveplate and a polarizer. (b) Nonlinear intensity modulation
Figure 3.Read out images of binary masks and resolution test charts. (a) Binary masks include a series of letters, “I ♡ N K U”. First row gives optical images of the masks captured directly using green light (532 nm). Images read by the red beam (820 nm) are shown in the second row. Scale bar is 10 μm. (b) Images of different sized resolution test charts by the green light are given in the first and third rows, while images by the read beam are given in the second and fourth rows. The sizes of chart images are labeled beside each pattern. The charts with sizes of 5 μm are well recognized as three distinct lines, corresponding to a spatial resolution of 500 lp/mm.
The spatial resolution is an important parameter of the SLM. The higher resolution refers to the finer modulation over the optical wavefront. The subwavelength sized unit cell not only makes the MS behave as a homogeneous film without optical diffraction, but also theoretically promises ultrahigh resolution with pixel sizes on the wavelength to subwavelength level. To assess the spatial resolution of the MS-OASLM, we replace the letter masks with resolution test charts, which consist of four sets of elements. Each element encloses three horizontal and three vertical lines. The charts are also fabricated by FIB milling through the opaque metal film. The resolution test charts are written onto the MS plane using the green light and form write images with sizes ranging from 20 to 5 μm, as shown in the first and third rows of Fig. 3(b). The readout images by the 820 nm light are given in the second and fourth rows. The elements with sizes of 5 μm are well recognized as three distinct lines without any blurring into one another. This implies a spatial resolution of about 500 lp/mm for our OASLM, and the corresponding single pixel size achieves about 1 μm. Such resolution is more than one order of magnitude higher than that of typical commercial SLM devices (for example, Hamamatsu X10468-01, 25 lp/mm) and at least two times larger than that of previously reported liquid crystal (LC)-based OASLMs [2,24,36].
3. CONCLUSION
In conclusion, we demonstrate here a novel ultracompact OASLM based on the nonlinear MS, which fulfills light modulation directly by another write light. Thanks to the outstanding performance of the MS in manipulating light via enhanced nonlinearities at nanoscales, the MS-OASLM acquires a merit of unprecedented compactness with a thickness of about 400 nm, which is less than one tenth of the thickness of the traditional LC-based SLMs. The subwavelength features of the MS make the MS-OASLM free of diffraction in the readout light. In addition, because no LC, electrode, and photosensing layer are needed, our MS-OASLM has a much simpler structure than the traditional LC-SLMs, and can be fabricated simply by standard lithography and spin-coating techniques. Based on our MS-OASLM, we demonstrate the image projection with a high resolution of up to 500 lp/mm, which is more than one order of magnitude higher than typical commercial SLM devices (for example, Hamamatsu X10468-01, 25 lp/mm). Furthermore, such MS-SLMs show advantages of flexibility, i.e., the spectral response of the nanostructures, and hence the read light wavelength of the MS-OASLM, could be easily tuned to any desired spectral range by changing the geometric parameters of the nanostructures. By replacing the traditional bulky optical elements in the projection system with state-of-the-art meta-components, such as metalenses [37], meta-polarizers [38], and meta-waveplates [39], the profile of the entire image projection system can be further greatly reduced. Such MS-based OASLMs could find wide applications in novel optical displays and all-optical data parallel processing techniques.
Acknowledgment
Acknowledgment. We thank the Nanofabrication Platform of Nankai University for fabricating the samples.
References
[1] J. W. Goodman. Introduction to Fourier Optics(1968).
[2] D. L. White, M. Feldman. Liquid-crystal light valves. Electron. Lett., 6, 837-839(1970).
[3] D. Casasent. Optical Data Processing: Applications(1978).
[4] U. Efron. Spatial Light Modulator Technology: Materials, Devices, and Applications(1994).
[5] N. Savage. Digital spatial light modulators. Nat. Photonics, 3, 170-172(2009).
[6] D. Vettese. Microdisplays: liquid crystal on silicon. Nat. Photonics, 4, 752-754(2010).
[7] A. Maimone, A. Georgiou, J. S. Kollin. Holographic near-eye displays for virtual and augmented reality. ACM Trans. Graph., 36, 85(2017).
[8] J. A. Christian, S. Cryan. A survey of lidar technology and its use in spacecraft relative navigation. AIAA Guidance, Navigation, and Control (GNC) Conference, 4641(2013).
[9] Y. M. Sigal, R. Zhou, X. Zhuang. Visualizing and discovering cellular structures with super-resolution microscopy. Science, 361, 880-887(2018).
[10] G. Moddel, P. R. Barbier. Spatial light modulators: processing light in real time. Opt. Photon. News, 8, 17-21(1997).
[11] P. K. Shrestha, Y. T. Chun, D. Chu. A high-resolution optically addressed spatial light modulator based on ZnO nanoparticles. Light Sci. Appl., 4, e259(2015).
[12] P. Chen, L.-L. Ma, W. Hu, Z.-X. Shen, H. K. Bisoyi, S.-B. Wu, S.-J. Ge, Q. Li, Y.-Q. Lu. Chirality invertible superstructure mediated active planar optics. Nat. Commun., 10, 2518(2019).
[13] S. Smith. Lasers, nonlinear optics and optical computers. Nature, 316, 319-324(1985).
[14] J. Zhang, H. Wang, S. Yoshikado, T. Aruga. Incoherent-to-coherent conversion by use of the photorefractive fanning effect. Opt. Lett., 22, 1612-1614(1997).
[15] M. Shih, A. Shishido, I. Khoo. All-optical image processing by means of a photosensitive nonlinear liquid-crystal film: edge enhancement and image addition-subtraction. Opt. Lett., 26, 1140-1142(2001).
[16] D. Woods, T. J. Naughton. Optical computing: photonic neural networks. Nat. Phys., 8, 257-259(2012).
[17] A. Solodar, T. A. Kumar, G. Sarusi, I. Abdulhalim. Infrared to visible image up-conversion using optically addressed spatial light modulator utilizing liquid crystal and InGaAs photodiodes. Appl. Phys. Lett., 108, 021103(2016).
[18] T. Beard, W. Bleha, S.-Y. Wong. ac liquid-crystal light valve. Appl. Phys. Lett., 22, 90-92(1973).
[19] J. Xu, G. Zhang, Q. Wu, Y. Liang, S. Liu, Q. Sun, X. Chen, Y. Shen. Holographic recording and light amplification in doped polymer film. Opt. Lett., 20, 504-506(1995).
[20] A. Grunnet-Jepsen, C. L. Thompson, W. E. Moerner. Spontaneous oscillation and self-pumped phase conjugation in a photorefractive polymer optical amplifier. Science, 277, 549-552(1997).
[21] L. Hesselink, S. S. Orlov, A. Liu, A. Akella, D. Lande, R. R. Neurgaonkar. Photorefractive materials for nonvolatile volume holographic data storage. Science, 282, 1089-1094(1998).
[22] A. Goonesekera, D. Wright, W. E. Moerner. Image amplification and novelty filtering with a photorefractive polymer. Appl. Phys. Lett., 76, 3358-3360(2000).
[23] Z. Yaqoob, D. Psaltis, M. S. Feld, C. Yang. Optical phase conjugation for turbidity suppression in biological samples. Nat. Photonics, 2, 110-115(2008).
[24] K. Miri Gelbaor, K. Matvey, L. Victor, C. Neil, I. Abdulhalim. Liquid crystal high-resolution optically addressed spatial light modulator using a nanodimensional chalcogenide photosensor. Opt. Lett., 39, 2048-2051(2014).
[25] S.-Q. Li, X. Xu, R. M. Veetil, V. Valuckas, R. Paniagua-Domnguez, A. I. Kuznetsov. Phase-only transmissive spatial light modulator based on tunable dielectric metasurface. Science, 364, 1087-1090(2019).
[26] J. Li, P. Yu, S. Zhang, N. Liu. Electrically-controlled digital metasurface device for light projection displays. Nat. Commun., 11, 3574(2020).
[27] N. I. Zheludev, Y. S. Kivshar. From metamaterials to metadevices. Nat. Mater., 11, 917-924(2012).
[28] M. Kauranen, A. V. Zayats. Nonlinear plasmonics. Nat. Photonics, 6, 737-748(2012).
[29] G. Li, S. Zhang, T. Zentgraf. Nonlinear photonic metasurfaces. Nat. Rev. Mater., 2, 17010(2017).
[30] X. Duan, S. Kamin, N. Liu. Dynamic plasmonic colour display. Nat. Commun., 8, 14606(2017).
[31] M. Ren, W. Cai, J. Xu. Tailorable dynamics in nonlinear optical metasurfaces. Adv. Mater., 32, 1806317(2020).
[32] J. Park, B. G. Jeong, S. I. Kim, D. Lee, J. Kim, C. Shin, C. B. Lee, T. Otsuka, J. Kyoung, S. Kim, K.-Y. Yang, Y.-Y. Park, J. Lee, I. Hwang, J. Jang, S. H. Song, M. L. Brongersma, K. Ha, S.-W. Hwang, H. Choo, B. L. Choi. All-solid-state spatial light modulator with independent phase and amplitude control for three-dimensional lidar applications. Nat. Nanotechnol., 16, 69-76(2020).
[33] M. Ren, M. Chen, W. Wu, L. Zhang, J. Liu, B. Pi, X. Zhang, Q. Li, S. Fan, J. Xu. Linearly polarized light emission from quantum dots with plasmonic nanoantenna arrays. Nano Lett., 15, 2951-2957(2015).
[34] M.-X. Ren, W. Wu, W. Cai, B. Pi, X.-Z. Zhang, J.-J. Xu. Reconfigurable metasurfaces that enable light polarization control by light. Light Sci. Appl., 6, e16254(2017).
[35] T. Lückemeyer, H. Franke. Nonlinear and bistable properties of doped PMMA lightguides. Appl. Phys. A, 55, 41-48(1992).
[36] S. Fukushima, T. Kurokawa, M. Ohno. Real-time hologram construction and reconstruction using a high-resolution spatial light modulator. Appl. Phys. Lett., 58, 787-789(1991).
[37] M. Khorasaninejad, W. T. Chen, R. C. Devlin, J. Oh, A. Y. Zhu, F. Capasso. Metalenses at visible wavelengths: diffraction-limited focusing and subwavelength resolution imaging. Science, 352, 1190-1194(2016).
[38] Y. Chen, J. Gao, X. Yang. Direction-controlled bifunctional metasurface polarizers. Laser Photon. Rev., 12, 1800198(2018).
[39] Y. Zhao, A. Alu. Tailoring the dispersion of plasmonic nanorods to realize broadband optical meta-waveplates. Nano Lett., 13, 1086-1091(2013).
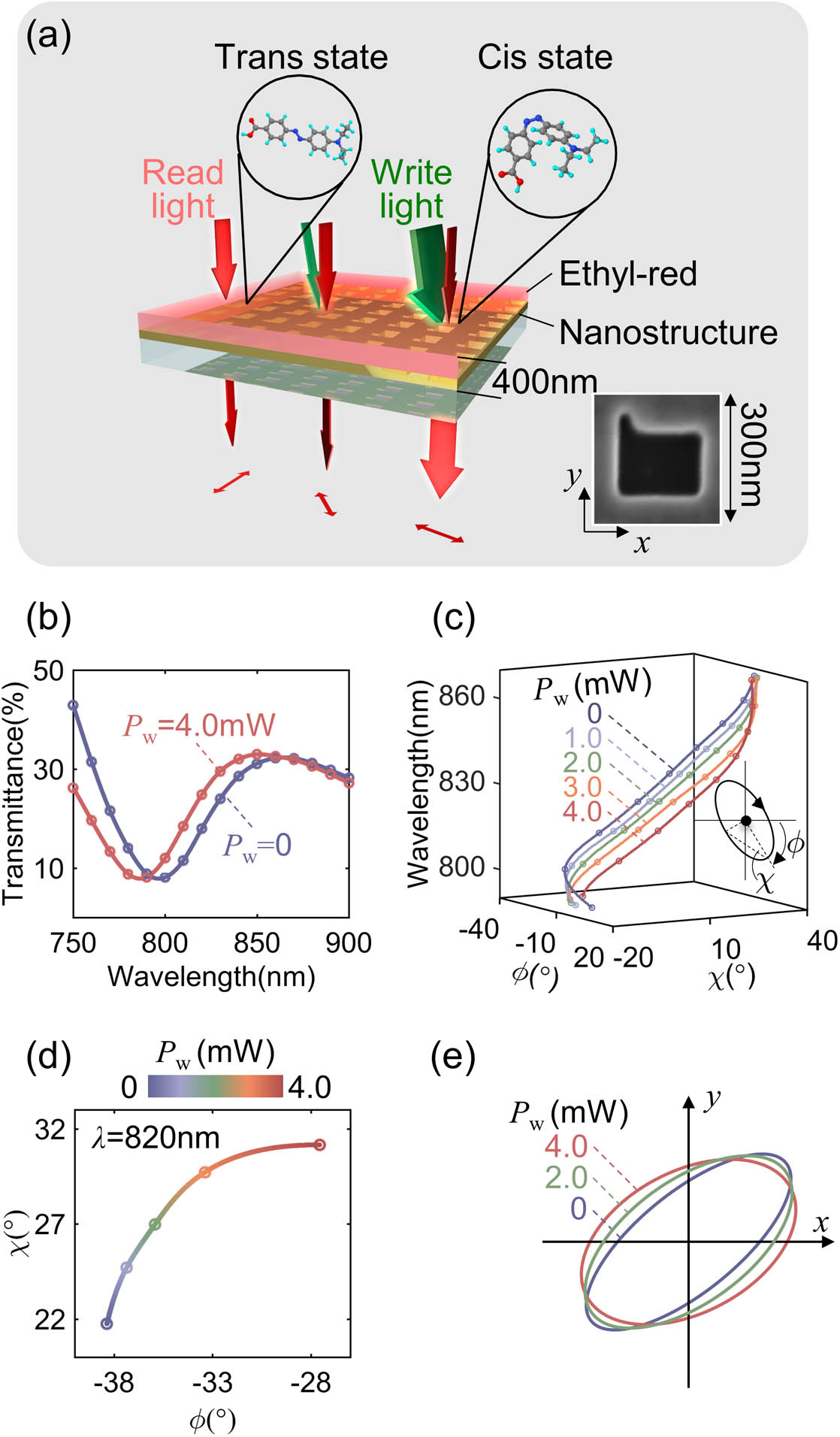
Set citation alerts for the article
Please enter your email address