Yijing Huang, Zhibo Dang, Xiao He, Zheyu Fang, "Engineering of single-photon emitters in hexagonal boron nitride [Invited]," Chin. Opt. Lett. 20, 032701 (2022)

Search by keywords or author
- Chinese Optics Letters
- Vol. 20, Issue 3, 032701 (2022)
![Measurement of quantum emission and structures of hBN. (a) Simple energy level diagram for quantum emission. When a quantum system is excited by light or electricity, the energy conversion between the excited state and the ground state can produce a single photon. (b), (c) The top-down and side views illustrating the in-plane honeycomb structure of hBN as well as the interlayer distance and stacking mode. B atoms and nitrogen atoms are represented in green and gray spheres. Within the (0001) plane, each N atom is threefold coordinated to B and vice versa in a honeycomb pattern that is a result of sp2 bonding. Out of the plane, hBN exhibits AA’-type stacking with alternating B and N atoms along with the [0001] direction. The interlayer separation is determined by the van der Waals interaction[15]. Copyright 2018, American Physical Society. (d) Charge-state transition levels for native point defects in hBN[15]. Copyright 2018, American Physical Society.](/richHtml/col/2022/20/3/032701/img_001.jpg)
Fig. 1. Measurement of quantum emission and structures of hBN. (a) Simple energy level diagram for quantum emission. When a quantum system is excited by light or electricity, the energy conversion between the excited state and the ground state can produce a single photon. (b), (c) The top-down and side views illustrating the in-plane honeycomb structure of hBN as well as the interlayer distance and stacking mode. B atoms and nitrogen atoms are represented in green and gray spheres. Within the (0001) plane, each N atom is threefold coordinated to B and vice versa in a honeycomb pattern that is a result of sp2 bonding. Out of the plane, hBN exhibits AA’-type stacking with alternating B and N atoms along with the [0001] direction. The interlayer separation is determined by the van der Waals interaction[15]. Copyright 2018, American Physical Society. (d) Charge-state transition levels for native point defects in hBN[15]. Copyright 2018, American Physical Society.
![Optical characterization of SPEs in hBN. (a) PL spectrum from hBN on different substrates. Each sample used CW excitation light at 532 nm. Although substrates may change the peaks of the PL spectrum, significant quantum emission can be observed[30]. Copyright 2016, American Chemical Society. (b) Confocal fluorescence images of suspended hBN films. The SE1–SE5 positions are marked. The dotted line represents the edge of the suspended sheet[31]. Copyright 2017, American Chemical Society. (c) PL spectra of SPEs in suspended hBN. Each row corresponds to different SPEs in SE1–SE5 positions[31]. Copyright 2017, ACS Nano. (d) CL spectrum acquired by integrating the recorded hyperspectral image from an individual hBN flake[32]. Copyright 2016, American Chemical Society.](/richHtml/col/2022/20/3/032701/img_002.jpg)
Fig. 2. Optical characterization of SPEs in hBN. (a) PL spectrum from hBN on different substrates. Each sample used CW excitation light at 532 nm. Although substrates may change the peaks of the PL spectrum, significant quantum emission can be observed[30]. Copyright 2016, American Chemical Society. (b) Confocal fluorescence images of suspended hBN films. The SE1–SE5 positions are marked. The dotted line represents the edge of the suspended sheet[31]. Copyright 2017, American Chemical Society. (c) PL spectra of SPEs in suspended hBN. Each row corresponds to different SPEs in SE1–SE5 positions[31]. Copyright 2017, ACS Nano. (d) CL spectrum acquired by integrating the recorded hyperspectral image from an individual hBN flake[32]. Copyright 2016, American Chemical Society.
![Schematics of optimized CVD growth methods. (a) Schematics of low-pressure CVD setup and hBN film on Cu[24]. Copyright 2019, American Chemical Society. (b) Schematic of atmospheric pressure CVD of hBN on Cu foil resting on quartz (Cu/quartz) or Ni (Cu/Ni) support substrate. The “standard” samples consisted of a piece of Cu foil being placed on a quartz backing, and the “gettered” samples consisted of a piece of Cu foil, which was backed with a piece of Ni foil. Quartz as the inert control substrate and Ni as the absorbent substrate have different effects on Cu. During the growth of CVD, the Ni is used as a B getter substrate on the back of the Cu foil. A gettering mechanism is proposed that B atoms preferentially diffuse into the Ni foil rather than into the Cu or remaining on the backside, as the precursor accumulates at this interface, namely the backside B gettering (BBG) process[44]. Copyright 2019, Wiley-VCH. (c) Diagram of integrated CVD growth and transfer process[45]. Copyright 2019, American Chemical Society. (d) Schematic illustration of SPEs localized by silica pillars[46]. Copyright 2021, American Chemical Society.](/Images/icon/loading.gif)
Fig. 3. Schematics of optimized CVD growth methods. (a) Schematics of low-pressure CVD setup and hBN film on Cu[24]. Copyright 2019, American Chemical Society. (b) Schematic of atmospheric pressure CVD of hBN on Cu foil resting on quartz (Cu/quartz) or Ni (Cu/Ni) support substrate. The “standard” samples consisted of a piece of Cu foil being placed on a quartz backing, and the “gettered” samples consisted of a piece of Cu foil, which was backed with a piece of Ni foil. Quartz as the inert control substrate and Ni as the absorbent substrate have different effects on Cu. During the growth of CVD, the Ni is used as a B getter substrate on the back of the Cu foil. A gettering mechanism is proposed that B atoms preferentially diffuse into the Ni foil rather than into the Cu or remaining on the backside, as the precursor accumulates at this interface, namely the backside B gettering (BBG) process[44]. Copyright 2019, Wiley-VCH. (c) Diagram of integrated CVD growth and transfer process[45]. Copyright 2019, American Chemical Society. (d) Schematic illustration of SPEs localized by silica pillars[46]. Copyright 2021, American Chemical Society.
![Engineering of quantum emitters in hBN. (a) Confocal map of the irradiated zone on a high-purity hBN flake of about 15 µm × 20 µm and 60 nm thickness, with eight irradiation spots (orange dashed lines)[48]. Copyright 2021, All Rights Reserved. (b) PL spectra from hBN flakes implanted with B, BN, O, and Si ions. The g2(τ) functions demonstrate that the emitters are single-photon sources[33]. Copyright 2016, American Chemical Society. (c) The linear defect density increases linearly with the plasma power. For fair comparison, the average linear density of emitters per edge length ρ = N/L is defined, since the defects were produced mostly at the edges of the flakes. Therefore, a crystal with the same area, but larger boundaries (so the area is distributed differently), can host more emitters, independently of the plasma parameters. The plasma time was 1 min[49]. Copyright 2018, American Chemical Society. (d) The linear defect density remains approximately constant when varying plasma times, at a constant plasma power of 100 W[49]. Copyright 2018, American Chemical Society. (e) Corresponding optical emission spectra to location P1 and spectral trajectory of type II emission in hBN. Spectral trajectories have been taken for the type II emission for the cases where hBN is located directly on a SiO2 substrate and located on top of an ALD-grown Al2O3 spacer layer with a thickness of 2 nm[50]. Copyright 2017, American Chemical Society. (f) A focused ion beam (FIB) is used to mill an array of patterned holes into hBN. Confocal spectra for quantum emitters made with various FIB parameters[51]. Copyright 2019, American Chemical Society.](/Images/icon/loading.gif)
Fig. 4. Engineering of quantum emitters in hBN. (a) Confocal map of the irradiated zone on a high-purity hBN flake of about 15 µm × 20 µm and 60 nm thickness, with eight irradiation spots (orange dashed lines)[48]. Copyright 2021, All Rights Reserved. (b) PL spectra from hBN flakes implanted with B, BN, O, and Si ions. The g2(τ) functions demonstrate that the emitters are single-photon sources[33]. Copyright 2016, American Chemical Society. (c) The linear defect density increases linearly with the plasma power. For fair comparison, the average linear density of emitters per edge length ρ = N/L is defined, since the defects were produced mostly at the edges of the flakes. Therefore, a crystal with the same area, but larger boundaries (so the area is distributed differently), can host more emitters, independently of the plasma parameters. The plasma time was 1 min[49]. Copyright 2018, American Chemical Society. (d) The linear defect density remains approximately constant when varying plasma times, at a constant plasma power of 100 W[49]. Copyright 2018, American Chemical Society. (e) Corresponding optical emission spectra to location P1 and spectral trajectory of type II emission in hBN. Spectral trajectories have been taken for the type II emission for the cases where hBN is located directly on a SiO2 substrate and located on top of an ALD-grown Al2O3 spacer layer with a thickness of 2 nm[50]. Copyright 2017, American Chemical Society. (f) A focused ion beam (FIB) is used to mill an array of patterned holes into hBN. Confocal spectra for quantum emitters made with various FIB parameters[51]. Copyright 2019, American Chemical Society.
![Strain engineering of SPEs in hBN. (a) Experimental scheme used to apply strain to hBN flakes sitting onto a bendable polycarbonate (PC) beam clamped at one edge. When the vertical force is applied to the free side, the color shows the simulated strain strength along the length (top panel) and width (bottom panel) of the beam. When tensile strain is generated in the length direction (x axis), the compressive strain is generated in the beam width direction (y axis) due to the PC Poisson’s ratio of 0.37[28]. Copyright 2017, The Authors. (b) The curve shows the scaled energy shift as a function of applied strain to the bendable substrate for three emitters with different tunability of −3.1 meV/% (green), + 3.3 meV/% (yellow), and + 6 meV/% (red). Inset shows a sketch of a quadratic energy shift ΔE for the single-photon emission induced by intrinsic strain[28]. Copyright 2017, The Authors. (c) Schematic diagrams for the strain applied in monolayer (upper part) and bilayer (lower part) geometries and the pressure-dependent PL spectra of hBN flakes at low temperatures by using a diamond anvil cell device[60]. Copyright 2018, American Chemical Society. (d) Schematic illustration of hBN film displaying a defect wavefunction. In the experiments, the strain is applied exclusively in the intralayer domain[61]. Copyright 2020, Wiley-VCH. (e) A simplified model showing the ground-state (|g〉) and excited-state (|e〉) levels for an atomic defect in hBN[61]. Copyright 2020, Wiley-VCH. (f) A wet transfer using a ∼20-nm-thick flake of hBN on a nanostructured silica substrate[5]. Copyright 2018, Optical Society of America. (g) The relationship between the energies of the emitted single photon and the external forces imposed on the (7,0) NBVN at BNNT[62]. Copyright 2019, American Chemical Society.](/Images/icon/loading.gif)
Fig. 5. Strain engineering of SPEs in hBN. (a) Experimental scheme used to apply strain to hBN flakes sitting onto a bendable polycarbonate (PC) beam clamped at one edge. When the vertical force is applied to the free side, the color shows the simulated strain strength along the length (top panel) and width (bottom panel) of the beam. When tensile strain is generated in the length direction (x axis), the compressive strain is generated in the beam width direction (y axis) due to the PC Poisson’s ratio of 0.37[28]. Copyright 2017, The Authors. (b) The curve shows the scaled energy shift as a function of applied strain to the bendable substrate for three emitters with different tunability of −3.1 meV/% (green), + 3.3 meV/% (yellow), and + 6 meV/% (red). Inset shows a sketch of a quadratic energy shift ΔE for the single-photon emission induced by intrinsic strain[28]. Copyright 2017, The Authors. (c) Schematic diagrams for the strain applied in monolayer (upper part) and bilayer (lower part) geometries and the pressure-dependent PL spectra of hBN flakes at low temperatures by using a diamond anvil cell device[60]. Copyright 2018, American Chemical Society. (d) Schematic illustration of hBN film displaying a defect wavefunction. In the experiments, the strain is applied exclusively in the intralayer domain[61]. Copyright 2020, Wiley-VCH. (e) A simplified model showing the ground-state (|g〉) and excited-state (|e〉) levels for an atomic defect in hBN[61]. Copyright 2020, Wiley-VCH. (f) A wet transfer using a ∼20-nm-thick flake of hBN on a nanostructured silica substrate[5]. Copyright 2018, Optical Society of America. (g) The relationship between the energies of the emitted single photon and the external forces imposed on the (7,0) NBVN at BNNT[62]. Copyright 2019, American Chemical Society.
![Electrical control of SPEs in hBN. (a) Device of the Stark effect in hBN SPE at room temperature. A, B, C, and D (yellow) denote the four Au electrodes where voltages are applied to generate external electric fields[67]. Copyright 2019, American Chemical Society. (b) ZPL spectra of the hBN SPE as a function of the voltage applied to electrodes A and B (inset) with equal magnitude but opposite signs[67]. Copyright 2019, American Chemical Society. (c) Device schematic of multilayer hBN sandwiched by the top and bottom few-layer graphene and PL spectrum of the emitter shown in (b) measured at 10 K and room temperature. In the inset is the second-order correlation [g2(Δt)] function of the emitter showing single-photon purity of g2(0)=0.3[68]. Copyright 2018, American Chemical Society. (d) The center wavelength shift of the emitter at 10 K (red dots) and room temperature (blue dots) under varying electric fields[68]. Copyright 2018, American Chemical Society. (e) Schematic representation of the experiment. An hBN flake is located on an ITO-covered glass substrate[69]. Copyright 2019, American Physical Society. (f) Measured spectra and corresponding fits of the unshifted emission in blue (left peak) and shifted in orange (right peak), taken with an integration time of 2.5 s and binned to 1 nm bins[69]. Copyright 2019, American Physical Society. (g) hBN-ZPL spectra recorded while the electric-field strength between the tip and ITO layer is lowered and increased again (from left to right, in 1 V steps). Spectra are recorded while a voltage of 20 V is switched on and off[69]. Copyright 2019, American Physical Society.](/Images/icon/loading.gif)
Fig. 6. Electrical control of SPEs in hBN. (a) Device of the Stark effect in hBN SPE at room temperature. A, B, C, and D (yellow) denote the four Au electrodes where voltages are applied to generate external electric fields[67]. Copyright 2019, American Chemical Society. (b) ZPL spectra of the hBN SPE as a function of the voltage applied to electrodes A and B (inset) with equal magnitude but opposite signs[67]. Copyright 2019, American Chemical Society. (c) Device schematic of multilayer hBN sandwiched by the top and bottom few-layer graphene and PL spectrum of the emitter shown in (b) measured at 10 K and room temperature. In the inset is the second-order correlation [g2( t)] function of the emitter showing single-photon purity of g2 [68]. Copyright 2018, American Chemical Society. (d) The center wavelength shift of the emitter at 10 K (red dots) and room temperature (blue dots) under varying electric fields[68]. Copyright 2018, American Chemical Society. (e) Schematic representation of the experiment. An hBN flake is located on an ITO-covered glass substrate[69]. Copyright 2019, American Physical Society. (f) Measured spectra and corresponding fits of the unshifted emission in blue (left peak) and shifted in orange (right peak), taken with an integration time of 2.5 s and binned to 1 nm bins[69]. Copyright 2019, American Physical Society. (g) hBN-ZPL spectra recorded while the electric-field strength between the tip and ITO layer is lowered and increased again (from left to right, in 1 V steps). Spectra are recorded while a voltage of 20 V is switched on and off[69]. Copyright 2019, American Physical Society.
![Temperature dependence of SPEs in hBN. (a)–(d) Temperature-dependent energy shift, line width, lifetime, and relative intensity for two ZPLs centered at 575 nm (green triangles) and 682 nm (red circles). Each ZPL shows nearly identical behavior, though the ZPL at 682 nm decreases in intensity more rapidly as temperature is increased[70]. Copyright 2016, American Chemical Society. (e) Optical image of the chromium microcircuit made by EBL. Insets: zoomed-in optical images of portions of the microcircuit where hBN nanoflakes have been transferred (numbered circles 1–3) and corresponding PL confocal map[71]. Copyright 2020, American Chemical Society. (f) Temperature measurement for the three positions in (e) as the current through the circuit is increased. Temperatures are estimated from the calibrated shift in the emitters’ ZPL[71]. Copyright 2020, American Chemical Society.](/Images/icon/loading.gif)
Fig. 7. Temperature dependence of SPEs in hBN. (a)–(d) Temperature-dependent energy shift, line width, lifetime, and relative intensity for two ZPLs centered at 575 nm (green triangles) and 682 nm (red circles). Each ZPL shows nearly identical behavior, though the ZPL at 682 nm decreases in intensity more rapidly as temperature is increased[70]. Copyright 2016, American Chemical Society. (e) Optical image of the chromium microcircuit made by EBL. Insets: zoomed-in optical images of portions of the microcircuit where hBN nanoflakes have been transferred (numbered circles 1–3) and corresponding PL confocal map[71]. Copyright 2020, American Chemical Society. (f) Temperature measurement for the three positions in (e) as the current through the circuit is increased. Temperatures are estimated from the calibrated shift in the emitters’ ZPL[71]. Copyright 2020, American Chemical Society.
![Magnetic-field control of SPEs in hBN. (a) Illustration of the coordinate system for magnetic fields concerning the microscope objective and sample. β, in the x^-z^ plane, defines the angle of the magnetic field concerning the sample plane, and α (ε), in the x^-y^ plane, denotes the absorptive (emissive) dipole angle[73]. Copyright 2019, The Authors. (b) PL variation as a function of the relative orientation between the SPE’s optical dipoles and an in-plane B = 890 G[73]. Copyright 2019, The Authors.](/Images/icon/loading.gif)
Fig. 8. Magnetic-field control of SPEs in hBN. (a) Illustration of the coordinate system for magnetic fields concerning the microscope objective and sample. β, in the x z plane, defines the angle of the magnetic field concerning the sample plane, and α (ε), in the x y plane, denotes the absorptive (emissive) dipole angle[73]. Copyright 2019, The Authors. (b) PL variation as a function of the relative orientation between the SPE’s optical dipoles and an in-plane B = 890 G[73]. Copyright 2019, The Authors.
![Photoinduced modification of SPEs in hBN. (a) Schematic of the electrical PEO:LiClO4 device used to modulate the single-photon emission[24]. Copyright 2019, American Chemical Society. (b) Normalized PL spectra of SPEs at gate voltages noted displaying a shift of 15 nm. Each spectrum is collected for 10 s; the spectra are offset for clarity[24]. Copyright 2019, American Chemical Society. (c) Schematic of the BMIM-PF6 ionic liquid device[24]. Copyright 2019, American Chemical Society. (d) A gradual 17 nm redshift is observed by 200 µW, 532 nm CW illumination to the sample; there is no gate voltage applied to induce this effect. In this case, the electrons are liberated from the ionic liquid by the laser excitation alone using a λexc = 532 nm illumination source at 200 µW. The spectra are collected for 10 s each during a time-resolved PL measurement and offset for clarity. The time axis maps the collection time of the spectra displayed[24]. Copyright 2019, American Chemical Society. (e) A redshift of 15 nm from another SPE is observed under the same experiment condition. All spectra are collected at 1 s acquisition time, showing the rapid kinetics of the shift observed[24]. Copyright 2019, American Chemical Society. (f) Spatial maps of integrated PL emission (spectral range from 2.06 to 2.15 eV) under green (532 nm, 2.33 eV) only and green plus blue (450 nm, 2.76 eV) excitation. PLE spectra with blue CW excitation for PLE laser polarization parallel (black squares) and perpendicular (red circles) to the emission from ZPL1 for defect A. The inset to (f) shows a polar plot of the intensity of the one phonon sideband of defect A as a function of the excitation polarization angle when resonant with ZPL1 (2.167 eV, black squares) and ZPL2 (2.556 eV, red circles)[75]. Copyright 2020, American Chemical Society.](/Images/icon/loading.gif)
Fig. 9. Photoinduced modification of SPEs in hBN. (a) Schematic of the electrical PEO:LiClO4 device used to modulate the single-photon emission[24]. Copyright 2019, American Chemical Society. (b) Normalized PL spectra of SPEs at gate voltages noted displaying a shift of 15 nm. Each spectrum is collected for 10 s; the spectra are offset for clarity[24]. Copyright 2019, American Chemical Society. (c) Schematic of the BMIM-PF6 ionic liquid device[24]. Copyright 2019, American Chemical Society. (d) A gradual 17 nm redshift is observed by 200 µW, 532 nm CW illumination to the sample; there is no gate voltage applied to induce this effect. In this case, the electrons are liberated from the ionic liquid by the laser excitation alone using a λexc = 532 nm illumination source at 200 µW. The spectra are collected for 10 s each during a time-resolved PL measurement and offset for clarity. The time axis maps the collection time of the spectra displayed[24]. Copyright 2019, American Chemical Society. (e) A redshift of 15 nm from another SPE is observed under the same experiment condition. All spectra are collected at 1 s acquisition time, showing the rapid kinetics of the shift observed[24]. Copyright 2019, American Chemical Society. (f) Spatial maps of integrated PL emission (spectral range from 2.06 to 2.15 eV) under green (532 nm, 2.33 eV) only and green plus blue (450 nm, 2.76 eV) excitation. PLE spectra with blue CW excitation for PLE laser polarization parallel (black squares) and perpendicular (red circles) to the emission from ZPL1 for defect A. The inset to (f) shows a polar plot of the intensity of the one phonon sideband of defect A as a function of the excitation polarization angle when resonant with ZPL1 (2.167 eV, black squares) and ZPL2 (2.556 eV, red circles)[75]. Copyright 2020, American Chemical Society.
![Optical coupling systems between SPEs and plasmonic nanostructures. (a) PL confocal map and PL spectra of a flake containing SPEs (red circled). Inset, SEM image of part of the flake on top of the Au plasmonic lattice[77]. Copyright 2017, American Chemical Society. (b) Bright-field optical microscopy image of various Ag pillar arrays on a glass substrate; (B) same as in (A) but after the transfer of a 20-nm-thick sheet of hBN[78]. Copyright 2019, The Authors. (c) Schematic illustration of the movement of an Au sphere towards an SPE in hBN using an AFM tip and schematic illustration of the movement of the second Au sphere to the same hBN flake[79]. Copyright 2018, The Royal Society of Chemistry. (d) Confocal luminescence image of hBN defects and the dipolar antennas, positioned nearby a clean quartz cover glass, both plotted on the same intensity scale. Luminescence spectra on the same scale of both the hBN defect (blue) and antenna (orange). Photon-arrival histograms for the hBN defect (blue) and the nanoantenna emission (orange)[80]. Copyright 2020, American Chemical Society. (e) Schematic of the on-chip MDA device. Spectrally integrated PL intensity versus pump power for the uncoupled (blue data points) and coupled states (red data points). Inset, emitter intensity over time showing excellent temporal stability without blinking[81]. Copyright 2019, American Chemical Society.](/Images/icon/loading.gif)
Fig. 10. Optical coupling systems between SPEs and plasmonic nanostructures. (a) PL confocal map and PL spectra of a flake containing SPEs (red circled). Inset, SEM image of part of the flake on top of the Au plasmonic lattice[77]. Copyright 2017, American Chemical Society. (b) Bright-field optical microscopy image of various Ag pillar arrays on a glass substrate; (B) same as in (A) but after the transfer of a 20-nm-thick sheet of hBN[78]. Copyright 2019, The Authors. (c) Schematic illustration of the movement of an Au sphere towards an SPE in hBN using an AFM tip and schematic illustration of the movement of the second Au sphere to the same hBN flake[79]. Copyright 2018, The Royal Society of Chemistry. (d) Confocal luminescence image of hBN defects and the dipolar antennas, positioned nearby a clean quartz cover glass, both plotted on the same intensity scale. Luminescence spectra on the same scale of both the hBN defect (blue) and antenna (orange). Photon-arrival histograms for the hBN defect (blue) and the nanoantenna emission (orange)[80]. Copyright 2020, American Chemical Society. (e) Schematic of the on-chip MDA device. Spectrally integrated PL intensity versus pump power for the uncoupled (blue data points) and coupled states (red data points). Inset, emitter intensity over time showing excellent temporal stability without blinking[81]. Copyright 2019, American Chemical Society.
![Optical coupling systems based on SPEs in hBN. (a) Confocal fluorescence image of an array of hBN/Si3N4, 3.5-µm-diameter microdisk cavities upon 510 nm laser excitation[82]. Copyright 2020, The Authors. (b) Schematic of the on-chip integration of hBN quantum emitters with photonic crystal cavities from Si nitride (Si3N4). PL spectra without normalization showing PL enhancement of the emitter[83]. Copyright 2020, American Chemical Society. (c) The microcavity consists of a hemispherical and flat mirror (only two stacks shown on either side). The quantum emitter hosted by hBN emits light confocally with the excitation laser[84]. Copyright 2019, American Chemical Society. (d) Schematic diagram of a device coupling a quantum emitter to an optical fiber. The setup consists of a home-made beam scanning confocal microscope equipped with a spectrometer and avalanche photodiodes for photon correlation measurements. The hBN flakes are connected to a tapered optical fiber (colored blue) with a radius of 320 nm using a piezo-driven triaxial manipulator (xyz) fitted. The microscope uses an objective lens with an NA of 0.95. Raster scanning is realized by a scanning mirror assembly (SM). The emitter in hBN is excited using a pulse or CW laser with a wavelength of 532 nm and detected by the back focal plane (BFP) of the objective or a CCD camera for imaging the sample. Light detection (Det) is accomplished through interchangeable modules for recording intensity, spectrum, and intensity correlation[85]. Copyright 2017, American Chemical Society. (e) Schematic design of micro-resonator device. The planar resonator is composed of a macroscopic plane mirror and a glass fiber tip with a concave structure. The hBN film grown by CVD is placed on the plane mirror to form a hybrid quantum emitter fiber-cavity system[86]. Copyright 2021, The Authors.](/Images/icon/loading.gif)
Fig. 11. Optical coupling systems based on SPEs in hBN. (a) Confocal fluorescence image of an array of hBN/Si3N4, 3.5-µm-diameter microdisk cavities upon 510 nm laser excitation[82]. Copyright 2020, The Authors. (b) Schematic of the on-chip integration of hBN quantum emitters with photonic crystal cavities from Si nitride (Si3N4). PL spectra without normalization showing PL enhancement of the emitter[83]. Copyright 2020, American Chemical Society. (c) The microcavity consists of a hemispherical and flat mirror (only two stacks shown on either side). The quantum emitter hosted by hBN emits light confocally with the excitation laser[84]. Copyright 2019, American Chemical Society. (d) Schematic diagram of a device coupling a quantum emitter to an optical fiber. The setup consists of a home-made beam scanning confocal microscope equipped with a spectrometer and avalanche photodiodes for photon correlation measurements. The hBN flakes are connected to a tapered optical fiber (colored blue) with a radius of 320 nm using a piezo-driven triaxial manipulator (xyz) fitted. The microscope uses an objective lens with an NA of 0.95. Raster scanning is realized by a scanning mirror assembly (SM). The emitter in hBN is excited using a pulse or CW laser with a wavelength of 532 nm and detected by the back focal plane (BFP) of the objective or a CCD camera for imaging the sample. Light detection (Det) is accomplished through interchangeable modules for recording intensity, spectrum, and intensity correlation[85]. Copyright 2017, American Chemical Society. (e) Schematic design of micro-resonator device. The planar resonator is composed of a macroscopic plane mirror and a glass fiber tip with a concave structure. The hBN film grown by CVD is placed on the plane mirror to form a hybrid quantum emitter fiber-cavity system[86]. Copyright 2021, The Authors.
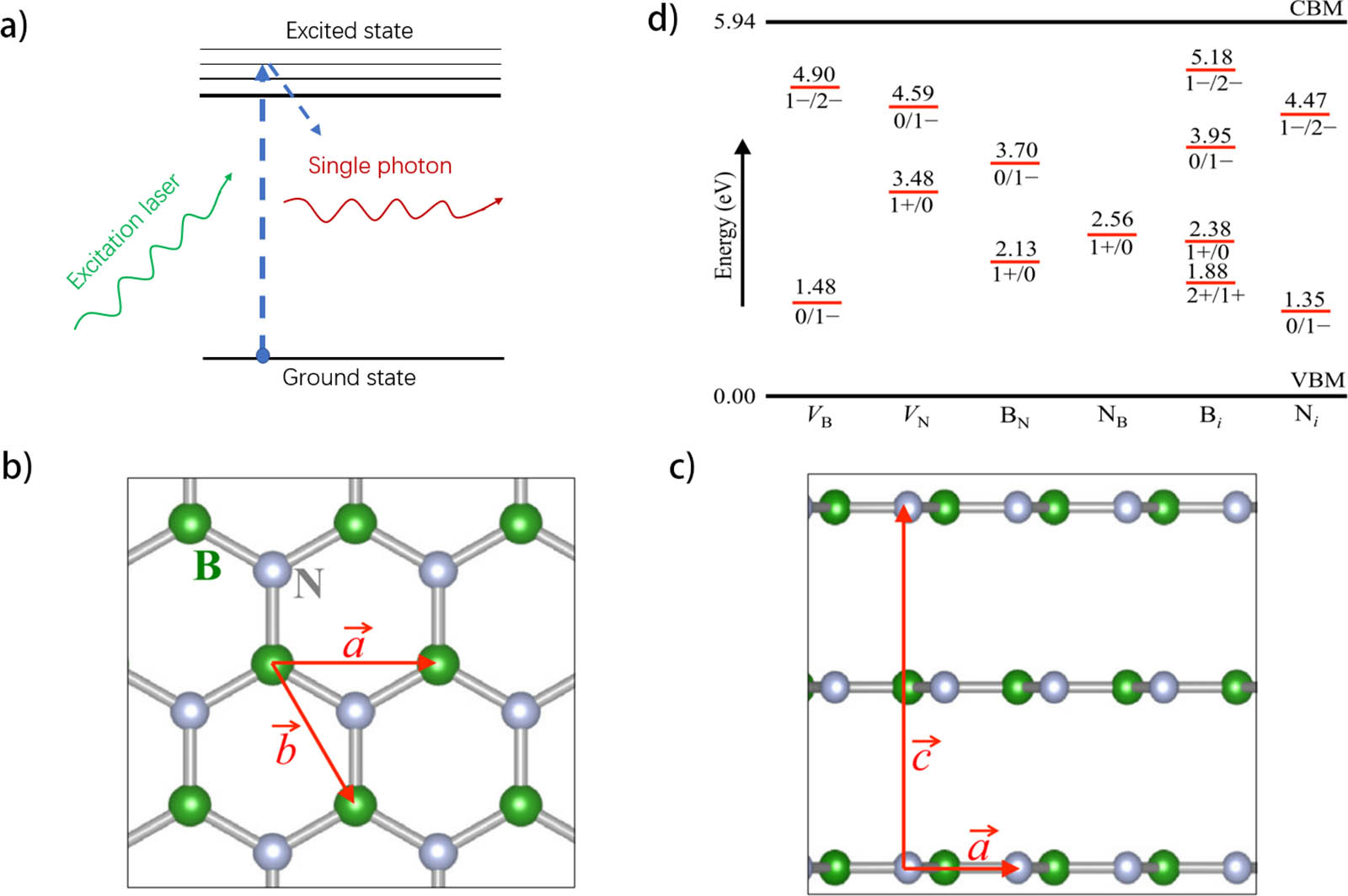
Set citation alerts for the article
Please enter your email address