
- Chinese Optics Letters
- Vol. 20, Issue 3, 032701 (2022)
Abstract
Keywords
1. Introduction
Over the past decades, the field of non-classical light sources has expanded exponentially, tightly related to the fields of quantum photonics, super-resolution imaging technology, and quantum information science, which specifically includes quantum key distribution, quantum computation, quantum communication, and precision sensing. In the experimental foundation of quantum mechanics[
Although there is still no ideal quantum emitter satisfying industrial production, quite a few quantum emitters in solid-state systems have been transformed from theoretical prediction to engineering design and optimized performance, such as color centers in crystals, quantum dots, carbon nanotubes, 2D materials, and other solid-state host materials[
Since the first observation of the quantum emission from hexagonal boron nitride (hBN) monolayers at room temperature[
Sign up for Chinese Optics Letters TOC. Get the latest issue of Chinese Optics Letters delivered right to you!Sign up now
In this review, research on the generation, properties, control, and applications of the quantum emitters in hBN is reviewed. Recent progress towards mechanism research of quantum emission in hBN is introduced. Multi-angle fabrication and coordination of SPEs in hBN are discussed. Next, the progress towards related applications is enumerated, respectively, such as integrating SPEs in hBN with photonic or plasmonic nanostructures, dielectric optical cavities, and waveguides. Lastly, the opportunities and challenges for SPEs in hBN are summarized, and new possibilities that are being explored at present and may be explored in the future are discussed.
2. Mechanism of Quantum Emission in hBN
The ideal SPE, as an isolated quantum system, that emits one photon at a time per excitation cycle needs to be satisfied, which is triggered optically or electrically[
Figure 1.Measurement of quantum emission and structures of hBN. (a) Simple energy level diagram for quantum emission. When a quantum system is excited by light or electricity, the energy conversion between the excited state and the ground state can produce a single photon. (b), (c) The top-down and side views illustrating the in-plane honeycomb structure of hBN as well as the interlayer distance and stacking mode. B atoms and nitrogen atoms are represented in green and gray spheres. Within the (0001) plane, each N atom is threefold coordinated to B and vice versa in a honeycomb pattern that is a result of sp2 bonding. Out of the plane, hBN exhibits AA’-type stacking with alternating B and N atoms along with the [0001] direction. The interlayer separation is determined by the van der Waals interaction[
In order to design high-quality quantum emitters in hBN, it is necessary to have a clear understanding of the atomic structure in hBN and the associated excitation processes that give rise to the quantum emission. hBN is a highly anisotropic crystal, consisting of B and N atoms strongly bonded in the in-plane direction. The B–N bond is polar covalent because of the difference in electronegativity between B and N[
2.1. 2 eV luminescence
When quantum emission at 623 nm from localized defects in hBN monolayers and multilayers at room temperature was first discovered, it showed extremely high brightness (
Density function theory (DFT) was used to investigate the origin of the defect responsible for the observed single-photon emission, by analyzing energy levels and the optical responses of the defects. In the beginning, the related study put forward that the
Figure 2.Optical characterization of SPEs in hBN. (a) PL spectrum from hBN on different substrates. Each sample used CW excitation light at 532 nm. Although substrates may change the peaks of the PL spectrum, significant quantum emission can be observed[
Some studies have shown that strain can induce modification of the optical characteristics of SPEs in hBN[
2.2. 4.1 eV luminescence
Using a custom-built CL + HBT system, a new extremely bright UV SPE (4.1 eV) in hBN[
To sum up, even though research into hBN SPEs has been expanding rapidly in the last decade, the defects causing these SPEs and the sources of the defects are still under debate. In particular, some new technologies, such as nanobeam electron diffraction[
3. Fabrication and Regulation of Quantum Emitters in hBN
As discussed before, hBN is considered a promising candidate for hosting bright, linearly polarized, and optically stable quantum emitters operating at room temperature and under harsh chemical conditions with high photon purity. However, the emission wavelength of fluorescence defects in hBN is uncontrolled yet, with ZPL energies widely distributed over a wide spectral range (hundreds of nanometers), hindering the potential development of related devices and applications. Besides, as they are located in atomically thin 2D materials, these SPEs have the unique potential to be solidly coupled to electronic and optoelectronic devices. To reliably integrate these SPEs into the hybrid devices, there is an urgent need for nanomanufacturing methods that control the generation and location of SPEs in hBN. In this section, different approaches to the fabrication and regulation of SPEs in hBN are introduced. On the one hand, growth techniques of hBN, such as CVD, are optimized, and a series of processing methods are employed, such as electron beam irradiation, annealing treatment, plasma etching, chemical etching, ion irradiation, proper surface passivation, laser processing, and UV ozone processing. On the other hand, external fields can be introduced for engineering SPEs in hBN, such as stress field, electrostatic field, and magnetic field.
3.1. Fabrication and optimization of SPEs in hBN
3.1.1. Chemical vapor deposition growth method
In a recent investigation of CVD, a bottom-up fabrication of hBN using low-pressure CVD (LPCVD) is proposed[
Figure 3.Schematics of optimized CVD growth methods. (a) Schematics of low-pressure CVD setup and hBN film on Cu[
Based on that, a further study puts forward an effective method to produce quantum emitters in hBN with the desired emission properties in different spectral regions by B diffusion through Cu via B during atmospheric pressure CVD (APCVD)[
Besides, a recent investigation demonstrated that the CVD process based on Pt catalyst can realize the controlled growth of large monolayer hBN and can also peel off the grown films directly from the catalyst to realize the cleaning process[
Another recent study demonstrates a positive approach to spatially control generation of hBN SPEs and provides an effective way to create large-scale SPE arrays[
3.1.2. Electron beam irradiation
The use of electron beam irradiation to fabricate and locate SPEs is attractive because this process does not require annealing and irradiation of 15 keV electrons in
Figure 4.Engineering of quantum emitters in hBN. (a) Confocal map of the irradiated zone on a high-purity hBN flake of about 15 µm × 20 µm and 60 nm thickness, with eight irradiation spots (orange dashed lines)[
3.1.3. Ion irradiation and neutron irradiation
Ion implantation was explored using B, B nitrogen (BN) complexes, silicon (Si), and O ions. B and BN were chosen to determine whether the probability of forming native defects would increase because these atoms mainly produce vacancies and interstitials. Si and O were selected to test whether the emission source is related to common foreign impurities such as O. Figure 4(b) shows the PL spectra of hBN flakes implanted with B, BN, O, and Si ions, respectively[
3.1.4. Plasma etching
The reported manufacturing techniques prove that O plasma etching can form color centers in the peeling multilayer hBN. To maximize the yield per flake of the SPEs and optimize the spectrum characteristics, plasma power and plasma time were changed. Figure 4(c) shows the functional relationship between the linear density per unit edge length and plasma power[
3.1.5. Chemical etching
Two methods of chemical etching are introduced here[
3.1.6. Proper surface passivation
A recent work explored a novel method based on atomic layer deposition (ALD) of a 2 nm thin
3.1.7. Focused ion beam
As mentioned above, SPEs tend to occur randomly at edges[
3.1.8. Ultraviolet ozone processing
The low-temperature ozone treatment also can be used to generate high-quality SPEs in hBN effectively. Ozone processing is an applicable low-temperature choice when some on-chip devices are not manufactured in a manner compatible with high-temperature annealing[
3.2. Introduced external fields to regulate the quantum emission of hBN
3.2.1. Strain engineering
Strain engineering is used to regulate the physical properties of materials by controlling the elastic strain fields applied to them[
Because of the strong in-plane atomic bonds in 2D materials, external strains can be applied to change the electronic energy levels of fluorescent defect states. For instance, the biaxial strain affects the electronic properties of carbon defects in hBN monolayers. When either B or nitrogen is replaced with a carbon atom, donor and acceptor states, respectively, are induced. By applying tensile strain and compression strain, the ionization energy of the donor and acceptor states can be controlled[
Bending of a flexible substrate is a common technique to induce strain. After post-growth processing (irradiation and annealing), hBN films are transferred onto a 1.5-mm-thick polycarbonate beam to control strain[
Figure 5.Strain engineering of SPEs in hBN. (a) Experimental scheme used to apply strain to hBN flakes sitting onto a bendable polycarbonate (PC) beam clamped at one edge. When the vertical force is applied to the free side, the color shows the simulated strain strength along the length (top panel) and width (bottom panel) of the beam. When tensile strain is generated in the length direction (x axis), the compressive strain is generated in the beam width direction (y axis) due to the PC Poisson’s ratio of 0.37[
Lately, new advances have been made in applying tensile strain to SPEs embedded in several layers of hBN films and achieving both red and blue spectral shifts with tuning amplitudes up to 65 meV[
In addition to tuning the ZPL spectrum of hBN, strain engineering can also be used to determinatively activate SPEs in the hBN[
It has been reported that the density of SPEs in hBN is related to the perimeter, so quasi-one-dimensional B-nitride nanotubes (BNNTs) with rich curvature have received much attention[
3.2.2. Acoustical modulation
Lately, the effect of surface acoustic waves (SAWs) on light emission from the defect center in the hBN was investigated[
3.2.3. Electrical control
The Stark effect, which describes the regulation of the emitted photon energy via shifting the electron energy level by an external electric field, is easily integrated into a quantum system, which has advantages over other tuning methods such as strain field. However, since most emission sources, such as quantum dots, atomic emitters such as
As mentioned above, due to high internal quantum efficiency, the hBN SPEs can work at room temperature with high brightness and light stability. Besides, the layered structure of the hBN may result in an in-plane dipole moment, which enables the applied in-plane electric field to be well aligned with the dipole direction. Based on that, a nanoscale four-electrode device is designed, which can not only control the direction of the electric field but also achieve an unprecedented in-plane field on the order of 0.1 V/nm, orders of magnitude higher than previous reports using a similar in-plane electrode design[
Figure 6.Electrical control of SPEs in hBN. (a) Device of the Stark effect in hBN SPE at room temperature. A, B, C, and D (yellow) denote the four Au electrodes where voltages are applied to generate external electric fields[
Besides, a method of effectively introducing electrostatic field and realizing large and reversible Stark-shift tuning of SPEs in hBN is introduced[
3.2.4. Temperature dependence
Figures 7(a)–7(d) show the temperature dependence of the spectral ZPLs caused by point defects in multilayer hBN. Although the ZPL transition energies differ by about 340 meV, they all redshift in a similar manner with temperature increase. The measured linewidth increases exponentially, and the relative strength of each ZPL decreases exponentially with temperature. A lattice vibration model that considers piezoelectric coupling to in-plane phonons can well explain these phenomena[
Figure 7.Temperature dependence of SPEs in hBN. (a)–(d) Temperature-dependent energy shift, line width, lifetime, and relative intensity for two ZPLs centered at 575 nm (green triangles) and 682 nm (red circles). Each ZPL shows nearly identical behavior, though the ZPL at 682 nm decreases in intensity more rapidly as temperature is increased[
Based on the sensitive temperature correlation in hBN, a deterministic optical temperature measurement technique based on SPEs in hBN is proposed in the subsequent research[
3.2.5. Magnetic field dependence
The magneto-optical effect is the main method to solve single spin and coupling to light. Electron paramagnetic resonance is known for bulk hBN[
Figure 8.Magnetic-field control of SPEs in hBN. (a) Illustration of the coordinate system for magnetic fields concerning the microscope objective and sample. β, in the x
3.2.6. Photoinduced modification
Two recent studies on optical tuning hBN quantum emission are presented. The work of optical doping by using ionic liquid devices is first introduced[
Figure 9.Photoinduced modification of SPEs in hBN. (a) Schematic of the electrical PEO:LiClO4 device used to modulate the single-photon emission[
4. Integration and Application Based on hBN
4.1. Photonic and plasmonic nanostructures
In the pioneering work of a hybrid quantum 2D material system integrated with plasmonic nanocavity arrays, the quantum emitters in the hBN were determinatively coupled to plasmonic Au and Ag arrays with high quality, and the coupled emitters showed better performance[
Figure 10.Optical coupling systems between SPEs and plasmonic nanostructures. (a) PL confocal map and PL spectra of a flake containing SPEs (red circled). Inset, SEM image of part of the flake on top of the Au plasmonic lattice[
4.2. Dielectric optical cavities and waveguides
In this section, the progress made in integrating the hBN SPEs with dielectric optical cavities and waveguides is briefly introduced. As shown in Fig. 11(a), an array of whispering gallery mode resonators in the form of Si nitride (
Figure 11.Optical coupling systems based on SPEs in hBN. (a) Confocal fluorescence image of an array of hBN/Si3N4, 3.5-µm-diameter microdisk cavities upon 510 nm laser excitation[
In addition to the short waveguides on the nano- and micro-scale mentioned above, the research on the integration of hBN emitters with optical fibers has made great progress in recent years, which is more important for remote applications such as quantum communication. The light emitted by an SPE into the optical fiber can be collected effectively, and it is proved that when the light is guided through the optical fiber, the quantum properties of light remain unchanged[
Recent progress of SPEs in an hBN monolayer provides a platform for optomechanical experiments in which SPEs can be coupled to the motion of a free-floating hBN membrane[
In addition to 2D material systems, there have been several studies looking at lower dimensional systems. For example, bright visible light SPEs have been found in a zero-dimensional B nitride allotrope (the B nitride nanococoon, BNNC)[
4.3. Future applications based on hBN
Besides the sensing experiments mentioned in Section 3.2 above, there is also some attractive work related to real quantum experiments[
Some of the fundamental principles of quantum theory, such as Born’s rule, are based on postulates that need to be tested experimentally. Any deviation from these postulates will show up in the results observed in experiments[
5. Conclusion
In this review, we focus on the progress of SPEs in hBN materials. The measurement method of quantum emission and the possible defects related to quantum emission in hBN are introduced. The origin of hBN quantum emission is still under debate. In actual scenarios, the heterogeneity of the hBN structure leads to the diversity of hBN defects, which makes it more difficult to explore the emission mechanism of hBN. As the basic unit of quantum information science, the regulation of hBN SPEs is a thriving field. From the two directions of various physical and chemical fabrication methods and introduction to the external field, the deterministic fabrication and effective engineering of hBN SPEs at the present stage are introduced in detail. In the future, more attention should be paid to how to adapt SPEs in hBN to large-scale or industrial applications with more accessible processes. On the technological side, methods need to be worked out that allow each irradiation point to be determined to obtain a single SPE[
References
[1] A. Peruzzo, P. Shadbolt, N. Brunner, S. Popescu, J. L. O’Brien. A quantum delayed-choice experiment. Science, 338, 634(2012).
[2] J. S. Lundeen, B. Sutherland, A. Patel, C. Stewart, C. Bamber. Direct measurement of the quantum wavefunction. Nature, 474, 188(2011).
[3] S. Castelletto, B. C. Johnson, V. Ivády, N. Stavrias, T. Umeda, A. Gali, T. Ohshima. A silicon carbide room-temperature single-photon source. Nat. Mater., 13, 151(2014).
[4] X. Liu, M. C. Hersam. 2D materials for quantum information science. Nat. Rev. Mater., 4, 669(2019).
[5] N. V. Proscia, Z. Shotan, H. Jayakumar, P. Reddy, C. Cohen, M. Dollar, A. Alkauskas, M. Doherty, C. A. Meriles, V. M. Menon. Near-deterministic activation of room-temperature quantum emitters in hexagonal boron nitride. Optica, 5, 1128(2018).
[6] I. Aharonovich, D. Englund, M. Toth. Solid-state single-photon emitters. Nat. Photonics, 10, 631(2016).
[7] T. T. Tran, K. Bray, M. J. Ford, M. Toth, I. Aharonovich. Quantum emission from hexagonal boron nitride monolayers. Nat. Nanotechnol., 11, 37(2016).
[8] A. K. Geim, I. V. Grigorieva. Van der Waals heterostructures. Nature, 499, 419(2013).
[9] K. S. Novoselov, A. Mishchenko, A. Carvalho, A. H. Castro Neto. 2D materials and van der Waals heterostructures. Science, 353, aac9439(2016).
[10] C. R. Dean, A. F. Young, I. Meric, C. Lee, L. Wang, S. Sorgenfrei, K. Watanabe, T. Taniguchi, P. Kim, K. L. Shepard, J. Hone. Boron nitride substrates for high-quality graphene electronics. Nat. Nanotechnol., 5, 722(2010).
[11] C. R. Woods, L. Britnell, A. Eckmann, R. S. Ma, J. C. Lu, H. M. Guo, X. Lin, G. L. Yu, Y. Cao, R. V. Gorbachev, A. V. Kretinin, J. Park, L. A. Ponomarenko, M. I. Katsnelson, Y. N. Gornostyrev, K. Watanabe, T. Taniguchi, C. Casiraghi, H. J. Gao, A. K. Geim, K. S. Novoselov. Commensurate-incommensurate transition in graphene on hexagonal boron nitride. Nat. Phys., 10, 451(2014).
[12] A. S. Mayorov, R. V. Gorbachev, S. V. Morozov, L. Britnell, R. Jalil, L. A. Ponomarenko, P. Blake, K. S. Novoselov, K. Watanabe, T. Taniguchi, A. K. Geim. Micrometer-scale ballistic transport in encapsulated graphene at room temperature. Nano Lett., 11, 2396(2011).
[13] L. Britnell, R. V. Gorbachev, R. Jalil, B. D. Belle, F. Schedin, A. Mishchenko, T. Georgiou, M. I. Katsnelson, L. Eaves, S. V. Morozov, N. M. R. Peres, J. Leist, A. K. Geim, K. S. Novoselov, L. A. Ponomarenko. Field-effect tunneling transistor based on vertical graphene heterostructures. Science, 335, 947(2012).
[14] J. D. Caldwell, I. Aharonovich, G. Cassabois, J. H. Edgar, B. Gil, D. N. Basov. Photonics with hexagonal boron nitride. Nat. Rev. Mater., 4, 552(2019).
[15] L. Weston, D. Wickramaratne, M. Mackoit, A. Alkauskas, C. G. Van de Walle. Native point defects and impurities in hexagonal boron nitride. Phys. Rev. B, 97, 214104(2018).
[16] M. Atatüre, D. Englund, N. Vamivakas, S.-Y. Lee, J. Wrachtrup. Material platforms for spin-based photonic quantum technologies. Nat. Rev. Mater., 3, 38(2018).
[17] S. D. C. Wehner, D. Elkouss Coronas, R. Hanson. Quantum internet: a vision for the road ahead. Science, 362, 303(2018).
[18] S. Castelletto, F. A. Inam, S. I. Sato, A. Boretti. Hexagonal boron nitride: a review of the emerging material platform for single-photon sources and the spin-photon interface. Beilstein. J. Nanotechnol., 11, 740(2020).
[19] M. Toth, I. Aharonovich. Single photon sources in atomically thin materials. Annu. Rev. Phys. Chem., 70, 123(2019).
[20] K. S. Novoselov, D. Jiang, F. Schedin, T. J. Booth, V. V. Khotkevich, S. V. Morozov, A. K. Geim. Two-dimensional atomic crystals. Proc. Natl. Acad. Sci. USA, 102, 10451(2005).
[21] J. N. Coleman, M. Lotya, A. O’Neill, S. D. Bergin, P. J. King, U. Khan, K. Young, A. Gaucher, S. De, R. J. Smith, I. V. Shvets, S. K. Arora, G. Stanton, H.-Y. Kim, K. Lee, G. T. Kim, G. S. Duesberg, T. Hallam, J. J. Boland, J. J. Wang, J. F. Donegan, J. C. Grunlan, G. Moriarty, A. Shmeliov, R. J. Nicholls, J. M. Perkins, E. M. Grieveson, K. Theuwissen, D. W. McComb, P. D. Nellist, V. Nicolosi. Two-dimensional nanosheets produced by liquid exfoliation of layered materials. Science, 331, 568(2011).
[22] M. Koperski, K. Nogajewski, M. Potemski. Single photon emitters in boron nitride: more than a supplementary material. Opt. Commun., 411, 158(2018).
[23] A. Hernandez-Minguez, J. Laehnemann, S. Nakhaie, J. M. J. Lopes, P. Santos. Luminescent defects in a few-layer h-BN film grown by molecular beam epitaxy. Phys. Rev. Appl., 10, 044031(2018).
[24] N. Mendelson, Z. Q. Xu, T. T. Tran, M. Kianinia, J. Scott, C. Bradac, I. Aharonovich, M. Toth. Engineering and tuning of quantum emitters in few-layer hexagonal boron nitride. ACS Nano, 13, 3132(2019).
[25] L. Schue, L. Sponza, A. Plaud, H. Bensalah, K. Watanabe, T. Taniguchi, F. Ducastelle, A. Loiseau, J. Barjon. Bright luminescence from indirect and strongly bound excitons in h-BN. Phys. Rev. Lett., 122, 067401(2019).
[26] F. Hayee, L. Yu, J. L. Zhang, C. J. Ciccarino, M. Nguyen, A. F. Marshall, I. Aharonovich, J. Vuckovic, P. Narang, T. F. Heinz, J. A. Dionne. Revealing multiple classes of stable quantum emitters in hexagonal boron nitride with correlated optical and electron microscopy. Nat. Mater., 19, 534(2020).
[27] T. T. Tran, C. Elbadawi, D. Totonjian, C. J. Lobo, G. Grosso, H. Moon, D. R. Englund, M. J. Ford, I. Aharonovich, M. Toth. Robust multicolor single photon emission from point defects in hexagonal boron nitride. ACS Nano, 10, 7331(2016).
[28] G. Grosso, H. Moon, B. Lienhard, S. Ali, D. K. Efetov, M. M. Furchi, P. Jarillo-Herrero, M. J. Ford, I. Aharonovich, D. Englund. Tunable and high-purity room temperature single-photon emission from atomic defects in hexagonal boron nitride. Nat. Commun., 8, 705(2017).
[29] P. Dev. Fingerprinting quantum emitters in hexagonal boron nitride using strain. Phys. Rev. Res., 2, 022050(2020).
[30] N. Chejanovsky, M. Rezai, F. Paolucci, Y. Kim, T. Rendler, W. Rouabeh, F. Favaro de Oliveira, P. Herlinger, A. Denisenko, S. Yang, I. Gerhardt, A. Finkler, J. H. Smet, J. Wrachtrup. Structural attributes and photodynamics of visible spectrum quantum emitters in hexagonal boron nitride. Nano Lett., 16, 7037(2016).
[31] A. L. Exarhos, D. A. Hopper, R. R. Grote, A. Alkauskas, L. C. Bassett. Optical signatures of quantum emitters in suspended hexagonal boron nitride. ACS Nano, 11, 3328(2017).
[32] R. Bourrellier, S. Meuret, A. Tararan, O. Stéphan, M. Kociak, L. H. G. Tizei, A. Zobelli. Bright UV single photon emission at point defects in h-BN. Nano Lett., 16, 4317(2016).
[33] S. Choi, T. T. Tran, C. Elbadawi, C. Lobo, X. Wang, S. Juodkazis, G. Seniutinas, M. Toth, I. Aharonovich. Engineering and localization of quantum emitters in large hexagonal boron nitride layers. ACS Appl. Mater. Interfaces, 8, 29642(2016).
[34] L. J. Martínez, T. Pelini, V. Waselowski, J. R. Maze, B. Gil, G. Cassabois, V. Jacques. Efficient single photon emission from a high-purity hexagonal boron nitride crystal. Phys. Rev. B, 94, 121405(2016).
[35] D. Yim, M. Yu, G. Noh, J. Lee, H. Seo. Polarization and localization of single-photon emitters in hexagonal boron nitride wrinkles. ACS Appl. Mater. Interfaces, 12, 36362(2020).
[36] N. Mendelson, M. Doherty, M. Toth, I. Aharonovich, T. T. Tran. Strain-induced modification of the optical characteristics of quantum emitters in hexagonal boron nitride. Adv. Mat., 32, 1908316(2020).
[37] N. Mendelson, D. Chugh, J. R. Reimers, T. S. Cheng, A. Gottscholl, H. Long, C. J. Mellor, A. Zettl, V. Dyakonov, P. H. Beton, S. V. Novikov, C. Jagadish, H. H. Tan, M. J. Ford, M. Toth, C. Bradac, I. Aharonovich. Identifying carbon as the source of visible single-photon emission from hexagonal boron nitride. Nat. Mater., 20, 321(2021).
[38] K. Era, F. Minami, T. Kuzuba. Fast luminescence from carbon-related defects of hexagonal boron nitride. J. Lumin., 24–25, 71(1981).
[39] M. R. Uddin, J. Li, J. Y. Lin, H. X. Jiang. Probing carbon impurities in hexagonal boron nitride epilayers. Appl. Phys. Lett., 110, 182107(2017).
[40] X. Z. Du, J. Li, J. Y. Lin, H. X. Jiang. The origin of deep-level impurity transitions in hexagonal boron nitride. Appl. Phys. Lett., 106, 021110(2015).
[41] T. Korona, M. Chojecki. Exploring point defects in hexagonal boron-nitrogen monolayers. Int. J. Quantum Chem., 119, e25925(2019).
[42] M. Mackoit-Sinkeviciene, M. Maciaszek, C. G. Van de Walle, A. Alkauskas. Carbon dimer defect as a source of the 4.1 eV luminescence in hexagonal boron nitride. Appl. Phys. Lett., 115, 212101(2019).
[43] C. Jara, T. Rauch, S. Botti, M. A. L. Marques, A. Norambuena, R. Coto, J. E. Castellanos-Aguila, J. R. Maze, F. Munoz. First-principles identification of single photon emitters based on carbon clusters in hexagonal boron nitride. J. Phys. Chem. A, 125, 1325(2021).
[44] I. H. Abidi, N. Mendelson, T. T. Tran, A. Tyagi, M. Zhuang, L. T. Weng, B. Özyilmaz, I. Aharonovich, M. Toth, Z. Luo. Selective defect formation in hexagonal boron nitride. Adv. Opt. Mater., 7, 1900397(2019).
[45] R. Wang, D. G. Purdie, Y. Fan, F. C. P. Massabuau, P. Braeuninger-Weimer, O. J. Burton, R. Blume, R. Schloegl, A. Lombardo, R. S. Weatherup, S. Hofmann. A peeling approach for integrated manufacturing of large monolayer h-BN crystals. ACS Nano, 13, 2114(2019).
[46] C. Li, N. Mendelson, R. Ritika, Y. Chen, Z.-Q. Xu, M. Toth, I. Aharonovich. Scalable and deterministic fabrication of quantum emitter arrays from hexagonal boron nitride. Nano Lett., 21, 3626(2021).
[47] N. Mendelson, L. Morales-Inostroza, C. Li, R. Ritika, M. A. P. Nguyen, J. Loyola-Echeverria, S. Kim, S. Götzinger, M. Toth, I. Aharonovich. Grain dependent growth of bright quantum emitters in hexagonal boron nitride. Adv. Opt. Mater., 9, 2001271(2021).
[48] C. Fournier, A. Plaud, S. Roux, A. Pierret, M. Rosticher, K. Watanabe, T. Taniguchi, S. Buil, X. Quélin, J. Barjon, J.-P. Hermier, A. Delteil. Position-controlled quantum emitters with reproducible emission wavelength in hexagonal boron nitride. Nat. Commun., 12, 3779(2021).
[49] T. Vogl, G. Campbell, B. C. Buchler, Y. Lu, P. K. Lam. Fabrication and deterministic transfer of high-quality quantum emitters in hexagonal boron nitride. ACS Photonics, 5, 2305(2018).
[50] X. Li, G. D. Shepard, A. Cupo, N. Camporeale, K. Shayan, Y. Luo, V. Meunier, S. Strauf. Nonmagnetic quantum emitters in boron nitride with ultranarrow and sideband-free emission spectra. ACS Nano, 11, 6652(2017).
[51] J. Ziegler, R. Klaiss, A. Blaikie, D. Miller, V. R. Horowitz, B. J. Aleman. Deterministic quantum emitter formation in hexagonal boron nitride via controlled edge creation. Nano Lett., 19, 2121(2019).
[52] J. R. Toledo, D. B. de Jesus, M. Kianinia, A. S. Leal, C. Fantini, L. A. Cury, G. A. M. Sáfar, I. Aharonovich, K. Krambrock. Electron paramagnetic resonance signature of point defects in neutron-irradiated hexagonal boron nitride. Phys. Rev. B, 98, 155203(2018).
[53] Z. Q. Xu, C. Elbadawi, T. T. Tran, M. Kianinia, X. Li, D. Liu, T. B. Hoffman, M. Nguyen, S. Kim, J. H. Edgar, X. Wu, L. Song, S. Ali, M. Ford, M. Toth, I. Aharonovich. Single photon emission from plasma treated 2D hexagonal boron nitride. Nanoscale, 10, 7957(2018).
[54] C. Li, Z.-Q. Xu, N. Mendelson, M. Kianinia, M. Toth, I. Aharonovich. Purification of single-photon emission from hBN using post-processing treatments. Nanophotonics, 8, 2049(2019).
[55] R. Roldan, A. Castellanos-Gomez, E. Cappelluti, F. Guinea. Strain engineering in semiconducting two-dimensional crystals. J. Phys. Condens. Matter, 27, 313201(2015).
[56] Y. Fujimoto, S. Saito. Band engineering and relative stabilities of hexagonal boron nitride bilayers under biaxial strain. Phys. Rev. B, 94, 245427(2016).
[57] C. Androulidakis, E. N. Koukaras, M. Poss, K. Papagelis, C. Galiotis, S. Tawfick. Strained hexagonal boron nitride: phonon shift and Grüneisen parameter. Phys. Rev. B, 97, 241414(2018).
[58] Y. Fujimoto, S. Saito. Effects of strain on carbon donors and acceptors in hexagonal boron nitride monolayers. Phys. Rev. B, 93, 045402(2016).
[59] O. Frank, G. Tsoukleri, I. Riaz, K. Papagelis, J. Parthenios, A. C. Ferrari, A. K. Geim, K. S. Novoselov, C. Galiotis. Development of a universal stress sensor for graphene and carbon fibres. Nat. Commun., 2, 255(2011).
[60] Y. Xue, H. Wang, Q. Tan, J. Zhang, T. Yu, K. Ding, D. Jiang, X. Dou, J. J. Shi, B. Q. Sun. Anomalous pressure characteristics of defects in hexagonal boron nitride flakes. ACS Nano, 12, 7127(2018).
[61] N. Mendelson, M. Doherty, M. Toth, I. Aharonovich, T. T. Tran. Strain-induced modification of the optical characteristics of quantum emitters in hexagonal boron nitride. Adv. Mater., 32, 1908316(2020).
[62] W. Hu, X. Cao, Y. Zhang, T. Li, J. Jiang, Y. Luo. Tunable single-photon emission by defective boron-nitride nanotubes for high-precision force detection. J. Phys. Chem. C, 123, 9624(2019).
[63] J. P. Perdew, K. Burke, M. Ernzerhof. Generalized gradient approximation made simple. Phys. Rev. Lett., 77, 3865(1996).
[64] N. Chejanovsky, Y. Kim, A. Zappe, B. Stuhlhofer, T. Taniguchi, K. Watanabe, D. Dasari, A. Finkler, J. H. Smet, J. Wrachtrup. Quantum light in curved low dimensional hexagonal boron nitride systems. Sci. Rep., 7, 14758(2017).
[65] L. Lindsay, D. A. Broido. Theory of thermal transport in multilayer hexagonal boron nitride and nanotubes. Phys. Rev. B, 85, 035436(2012).
[66] F. Iikawa, A. Hernández-Mínguez, I. Aharonovich, S. Nakhaie, Y.-T. Liou, J. M. J. Lopes, P. V. Santos. Acoustically modulated optical emission of hexagonal boron nitride layers. Appl. Phys. Lett., 114, 171104(2019).
[67] Y. Xia, Q. Li, J. Kim, W. Bao, C. Gong, S. Yang, Y. Wang, X. Zhang. Room-temperature giant Stark effect of single photon emitter in van der Waals material. Nano Lett., 19, 7100(2019).
[68] G. Noh, D. Choi, J. H. Kim, D. G. Im, Y. H. Kim, H. Seo, J. Lee. Stark tuning of single-photon emitters in hexagonal boron nitride. Nano Lett., 18, 4710(2018).
[69] N. Nikolay, N. Mendelson, N. Sadzak, F. Böhm, T. T. Tran, B. Sontheimer, I. Aharonovich, O. Benson. Very large and reversible Stark-shift tuning of single emitters in layered hexagonal boron nitride. Phys. Rev. Appl., 11, 041001(2019).
[70] N. R. Jungwirth, B. Calderon, Y. Ji, M. G. Spencer, M. E. Flatte, G. D. Fuchs. Temperature dependence of wavelength selectable zero-phonon emission from single defects in hexagonal boron nitride. Nano Lett., 16, 6052(2016).
[71] Y. Chen, T. N. Tran, N. M. H. Duong, C. Li, M. Toth, C. Bradac, I. Aharonovich, A. Solntsev, T. T. Tran. Optical thermometry with quantum emitters in hexagonal boron nitride. ACS Appl. Mater. Interfaces, 12, 25464(2020).
[72] H. Akbari, W.-H. Lin, B. Vest, P. K. Jha, H. A. Atwater. Temperature-dependent spectral emission of hexagonal boron nitride quantum emitters on conductive and dielectric substrates. Phys. Rev. Appl., 15, 014036(2021).
[73] A. L. Exarhos, D. A. Hopper, R. N. Patel, M. W. Doherty, L. C. Bassett. Magnetic-field-dependent quantum emission in hexagonal boron nitride at room temperature. Nat. Commun., 10, 222(2019).
[74] M. Fanciulli. Electron paramagnetic resonance and relaxation in BN and BN: C. Philos. Mag. B, 76, 363(1997).
[75] P. Khatri, A. J. Ramsay, R. N. E. Malein, H. M. H. Chong, I. J. Luxmoore. Optical gating of photoluminescence from color centers in hexagonal boron nitride. Nano Lett., 20, 4256(2020).
[76] Z. Shotan, H. Jayakumar, C. R. Considine, M. Mackoit, H. Fedder, J. Wrachtrup, A. Alkauskas, M. W. Doherty, V. M. Menon, C. A. Meriles. Photoinduced modification of single-photon emitters in hexagonal boron nitride. ACS Photonics, 3, 2490(2016).
[77] T. T. Tran, D. Wang, Z. Q. Xu, A. Yang, M. Toth, T. W. Odom, I. Aharonovich. Deterministic coupling of quantum emitters in 2D materials to plasmonic nanocavity arrays. Nano Lett., 17, 2634(2017).
[78] N. V. Proscia, R. J. Collison, C. A. Meriles, V. M. Menon. Coupling of deterministically activated quantum emitters in hexagonal boron nitride to plasmonic surface lattice resonances. Nanophotonics, 8, 2057(2019).
[79] M. Nguyen, S. Kim, T. T. Tran, Z. Q. Xu, M. Kianinia, M. Toth, I. Aharonovich. Nanoassembly of quantum emitters in hexagonal boron nitride and gold nanospheres. Nanoscale, 10, 2267(2018).
[80] N. Palombo Blascetta, M. Liebel, X. Lu, T. Taniguchi, K. Watanabe, D. K. Efetov, N. F. van Hulst. Nanoscale imaging and control of hexagonal boron nitride single photon emitters by a resonant nanoantenna. Nano Lett., 20, 1992(2020).
[81] X. Li, R. A. Scully, K. Shayan, Y. Luo, S. Strauf. Near-unity light collection efficiency from quantum emitters in boron nitride by coupling to metallo-dielectric antennas. ACS Nano, 13, 6992(2019).
[82] N. V. Proscia, H. Jayakumar, X. Ge, G. Lopez-Morales, Z. Shotan, W. Zhou, C. A. Meriles, V. M. Menon. Microcavity-coupled emitters in hexagonal boron nitride. Nanophotonics, 9, 2937(2020).
[83] J. E. Froch, S. Kim, N. Mendelson, M. Kianinia, M. Toth, I. Aharonovich. Coupling hexagonal boron nitride quantum emitters to photonic crystal cavities. ACS Nano, 14, 7085(2020).
[84] T. Vogl, R. Lecamwasam, B. C. Buchler, Y. Lu, P. K. Lam. Compact cavity-enhanced single-photon generation with hexagonal boron nitride. ACS Photonics, 6, 1955(2019).
[85] A. W. Schell, H. Takashima, T. T. Tran, I. Aharonovich, S. Takeuchi. Coupling quantum emitters in 2D materials with tapered fibers. ACS Photonics, 4, 761(2017).
[86] S. Häußler, G. Bayer, R. Waltrich, N. Mendelson, C. Li, D. Hunger, I. Aharonovich, A. Kubanek. Tunable fiber-cavity enhanced photon emission from defect centers in hBN. Adv. Opt. Mater., 9, 2002218(2021).
[87] Y. Chen, X. Xu, C. Li, A. Bendavid, M. T. Westerhausen, C. Bradac, M. Toth, I. Aharonovich, T. T. Tran. Bottom-up synthesis of hexagonal boron nitride nanoparticles with intensity-stabilized quantum emitters. Small, 17, 2008062(2021).
[88] M. Abdi, M. B. Plenio. Quantum effects in a mechanically modulated single-photon emitter. Phys. Rev. Lett., 122, 023602(2019).
[89] J. Ziegler, A. Blaikie, A. Fathalizadeh, D. Miller, F. S. Yasin, K. Williams, J. Mohrhardt, B. J. McMorran, A. Zettl, B. Aleman. Single-photon emitters in boron nitride nanococoons. Nano Lett., 18, 2683(2018).
[90] M. Herrero-Collantes, J. C. Garcia-Escartin. Quantum random number generators. Rev. Mod. Phys., 89, 015004(2017).
[91] T. Jennewein, U. Achleitner, G. Weihs, H. Weinfurter, A. Zeilinger. A fast and compact quantum random number generator. Rev. Sci. Instrum., 71, 1675(2000).
[92] M. Hoese, M. K. Koch, F. Breuning, N. Lettner, K. G. Fehler, A. Kubanek. Single photon randomness originating from the symmetry of dipole emission and the unpredictability of spontaneous emission(2021).
[93] R. D. Sorkin. Quantum mechanics as quantum measure theory. Mod. Phys. Lett. A, 09, 3119(1994).
[94] T. Kauten, R. Keil, T. Kaufmann, B. Pressl, Č. Brukner, G. Weihs. Obtaining tight bounds on higher-order interferences with a 5-path interferometer. New J. Phys., 19, 033017(2017).
[95] T. Vogl, H. Knopf, M. Weissflog, P. K. Lam, F. Eilenberger. Sensitive single-photon test of extended quantum theory with two-dimensional hexagonal boron nitride. Phys. Rev. Res., 3, 013296(2021).
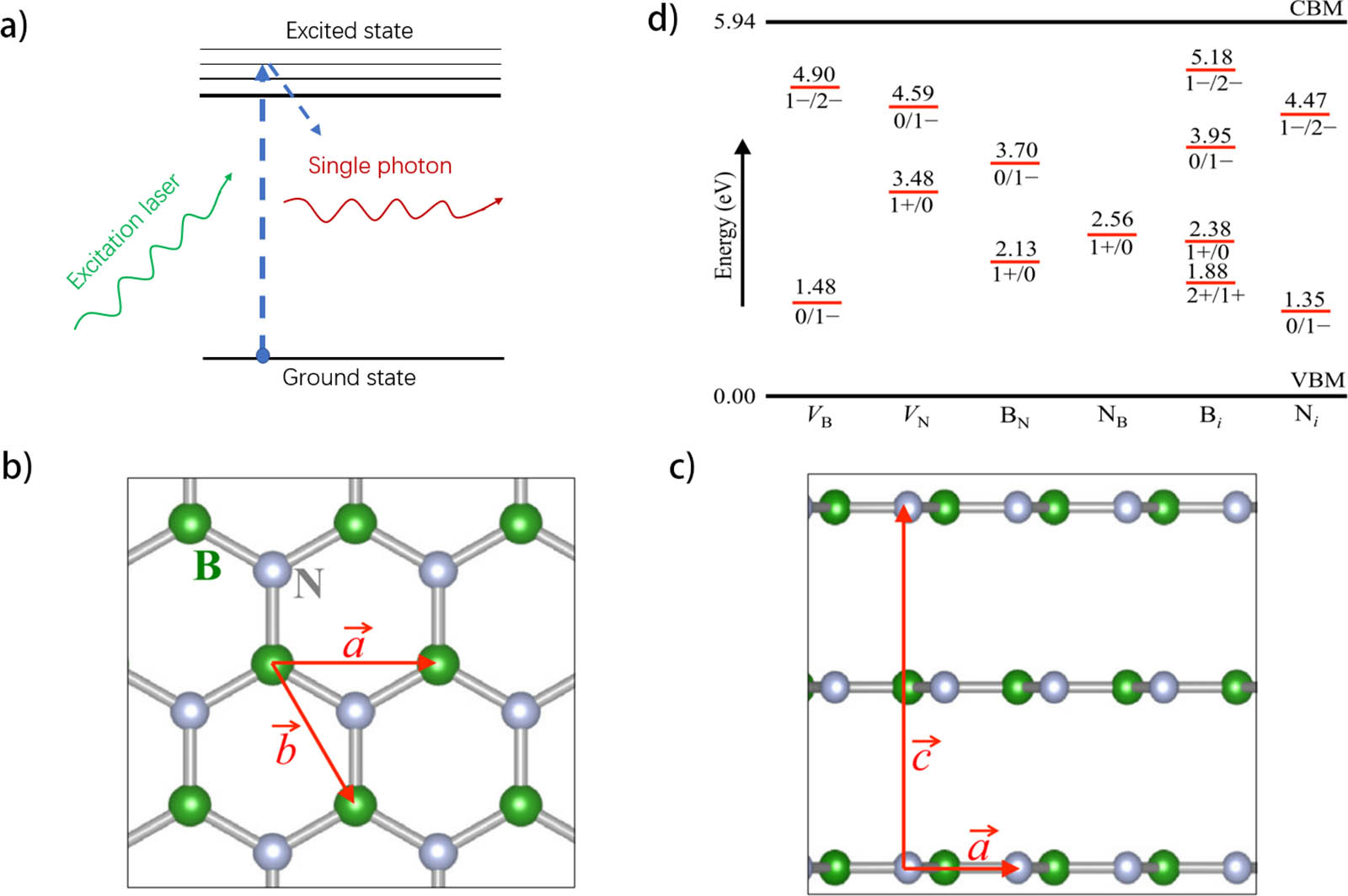
Set citation alerts for the article
Please enter your email address