
- Photonics Research
- Vol. 10, Issue 8, 1937 (2022)
Abstract
1. INTRODUCTION
Ultrafast imaging is extremely important for measuring unrepeatable transient phenomena, such as the propagation of shock wave [1], laser-induced damage [2], and exciton diffusion [3], which were difficult to measure with a common video camera. Compared to a traditional mechanical ultrafast camera that improves imaging speed by reducing the exposure time of detector [4–6], most ultrafast imaging techniques reconstruct a sequence of images from one frame of data recorded with single detector exposure [7–10]. Ultrafast imaging can generate both intensity and phase images depending on the optical alignment and the reconstruction algorithm applied [9,11–13] and, generally speaking, ultrafast phase imaging is more sensitive and suitable for observation of transparent dynamic samples such as laser plasma [13–15] and shock wave [16,17]. Compressed ultrafast photography (CUP) is the fastest intensity imaging technique used now. It applies a strategy of compressive sensing and can reach the speed of 10 trillion frames per second (Tfps) in imaging a sparse-enough sample [18–20]. Ultrafast phase imaging was commonly realized using the strategy of ultrafast interferometry [9], where multilaser pulses illuminate a sample sequentially and interfere with an additional reference beam. Then the interferogram formed was recorded with single detector exposure. When the polarization or incident angle of each illuminating pulse is different, the phase and amplitude of all laser pulses leaving the sample at slightly different times can be separately numerically reconstructed. The highest temporal resolution of this interferometry-based ultrafast phase imaging technique is 1.6 ns; however, the reference beam adopted makes the optical alignment of interferometry-based ultrafast phase imaging quite complex, which seriously limits its application. On the other hand, since the interferogram of each illuminating laser pulse occupies only a small region in spatial domain or frequency domain, the achieved spatial resolution is inversely proportional to the number of illuminating laser pulses.
Coherent diffraction imaging (CDI) was mainly developed for short wavelengths, including X-rays [21,22] and high energy electron beams [23,24], where high-quality optical elements are not available. CDI can reach diffraction-limited resolution via iterative reconstructing approaches [25,26]. The outstanding advantage of CDI lies in its capability to realize phase imaging with simple optical alignment, and the accuracy and image quality of CDI are now comparable to that of traditional interferometry and holography, even in the regime of visible light. Several researches have illustrated the possibility of using CDI to replace interferometry to realize ultrafast phase imaging with a compact optical structure [27,28]. However, since each recorded subdiffraction pattern occupies only a small region of the sensing chip, CDI-based ultrafast phase imaging suffers from degradation in the spatial resolution. In other words, time-resolved phase imaging was realized with CDI at the cost of low spatial resolution, reducing its applicability for circumstances that require both high spatial and temporal resolutions.
A multimode CDI algorithm [29–31] that takes full advantages of the information redundancy involved in diffraction intensity provides a new approach to circumvent the problem of degradation in the spatial resolution in CDI-based ultrafast phase imaging. This paper proposes a kind of single-shot ultrafast multiplexed CDI (SUM–CDI) to realize quantitative ultrafast phase imaging without degradation in the spatial resolution. Several laser pulses illuminate the sample under inspection in sequence, and diffraction pattern arrays formed by each laser pulse that arrive at the sensor chip at different times are recorded with a single detector exposure. Then, time-resolved images can be reconstructed using a specially designed multiplexed CDI algorithm. The temporal resolution of this proposed method was determined by the pulse duration of the illuminating pulses, which was changeable in a range from microseconds to femtoseconds. On the other hand, since the diffraction patterns formed by each illuminating laser pulse occupy the entire sensor chip of the detector, the reconstructed images have high-enough temporal and spatial resolutions. The performance of this proposed approach was verified by imaging the generation and evolution of laser-induced damage and the accompanying phenomenon of laser filament and shock wave inside K9 glass at a temporal resolution of 10 ns and a spatial resolution of 6.96 μm. The temporal resolution can be improved further to picoseconds or femtoseconds by using shorter laser pulses for illumination, and its spatial resolution can be improved further by using a larger sensor chip. To the best of our knowledge, this is the first time that single-shot ultrafast phase imaging with multiplexed CDI has been realized and used to experimentally measure unrepeatable transient process.
Sign up for Photonics Research TOC. Get the latest issue of Photonics Research delivered right to you!Sign up now
2. PRINCIPLE
The principle of the proposed SUM–CDI was schematically shown in Fig. 1(a), where several focused laser pulses illuminate a specimen at incident angles of
Figure 1.(a) Schematic diagram of the principle of SUM–CDI. (b) Data flow chart of SUM–CDI.
When there is only one illuminating pulse,
When a series of laser pulses are incident on a specimen at times of
Figure 1(b) shows the reconstructing data flow chart of this proposed method corresponding to Fig. 1(a). After giving two initial guesses to two images of
3. COMPUTATION METHOD
The computing method is shown in Fig. 2, where an initial guess was given to each probing laser pulse arriving at encoding plate as
Figure 2.Iterative computation method of SUM–CDI.
4. EXPERIMENTAL RESULTS
Figure 3.(a) Optical path diagram of the SUM–CDI experimental system. (b) Amplitude distribution of the encoding plate. (c) Phase distribution of the encoding plate. The units of the color bar of (c) are in radians. The scale bar in (b) is applicable to (c).
The temporal intervals among four probe laser pulses of 532 nm and one pump pulse of 352 nm are shown in Fig. 4(a), where the first probe pulse arrives at the specimen about 10.2 ns earlier than the pump pulse. The second probe pulse and the pump pulse arrive at the specimen simultaneously, and the pump pulse causes damage in K9 glass. The temporal intervals of the third probe pulse to the second and the fourth ones are 16.8 ns and 11.5 ns, respectively. Thus, the first probe reveals the original state of specimen, and the second probe reveals the state of the specimen during the interaction between the pump laser pulse and glass, and the third and the fourth pulses show the evaluation of damage after the finish of laser-material interaction. The temporal resolution of this suggested SUM–CDI in Fig. 3 was about 10 ns, and it depends on the pulse duration of the probe pulse. The energy density of pump pulse
Figure 4.(a) Time sequence diagram of probe pulse sequence and pump pulse in single-shot mode. (b) Intensity diagram of diffraction pattern in shot 1. (c) Amplitude distribution of the specimen at the corresponding time of each frame reconstructed by SUM–CDI. (d) Phase distribution of the specimen at the corresponding time of each frame reconstructed by SUM–CDI. The units of the color bar of phase in (d) are in radians. The scale bar in (c) is applicable to (d).
From the recorded diffraction patterns shown in Fig. 4(b), the intensity and phase of four transmitted probe pulses are reconstructed and shown in the first line of Fig. 4(c) and Fig. 4(d), respectively. K9 glass is uniform in both the refractive index and surface profile before the arrival of a pump pulse; thus, the reconstructed intensity and phase images corresponding to the initial state of specimen at the time of
To further check the correctness of measurements above, the speed of the shock wave was calculated using the experimental results above. The values of the reconstructed phase images corresponding to three pump pulses are plotted along the blue broken lines and shown in Figs. 5(a), 5(b), and 5(c), respectively. In Fig. 4(d), the 12 subfigures use one colorbar, and the background value is unified to the minimum value. Therefore, the phase range of the 1D curve in Fig. 5 is less than
Figure 5.Phase curves in (a) Shot 1, (b) Shot 2, and (c) Shot 3. The phase curves are plotted along blue broken lines in Fig.
Figure 6.Experimental result on spatial resolving capability. (a1)–(a4) Diffraction patterns recorded separately, and (a5) hybrid diffraction pattern by adding (a1)–(a4) together. (b1)–(b4) Images reconstructed from (a1)–(a4), respectively. (c1)–(c4) Reconstructed images from (a5) with SUM–CDI. (d1)–(d4) Resolution comparison between (c1)–(c4) and (b1)–(b4), respectively.
Figure 7.Experimental results on accuracy in phase object imaging. (a1)–(a4) Diffraction patterns recorded separately, and (a5) hybrid diffraction pattern by adding (a1)–(a4) together. (b1)–(b4) Images reconstructed from (a1)–(a4), respectively. (c1)–(c4) Reconstructed images from (a5) with SUM–CDI. (d1)–(d4) Accuracy comparison between (c1)–(c4) and (b1)–(b4), respectively. The units of the color bar are in radians.
5. DISCUSSION
Different from existing ultrafast phase imaging techniques, SUM–CDI applies a multiplexed strategy to avoid the division in the spatial domain or frequency domain, so it can achieve ultrafast phase imaging with high temporal–spatial resolutions and high accuracy by using a simpler optical setup. Theoretically, by using a detector with a large enough sensor chip and high enough dynamic range, diffraction limited spatial resolution can be reached, and its temporal resolution is only decided by the time duration of probing laser beams. Thus, by using a 32 bit detector, temporal–spatial resolution much higher than that of the experiments above is reachable.
6. CONCLUSION
To realize a sequence of ultrafast phase and amplitude imaging with a single diffraction pattern, a new scheme SUM–CDI that includes a grating, a weak modulator, a detector, and phase retrieval algorithms is proposed in this paper. Due to the information redundancy introduced by multiple modulation and the multiplex phase retrieval algorithm inspired by ptychography and CMI, at least four wave modes, including phase and amplitude, can be iteratively retrieved at once with a higher SNR. When combining with an ultrafast probe sequence, SUM–CDI could be used as an excellent technique for single-shot ultrafast optical imaging, which was demonstrated by measuring laser-induced damage with a temporal resolution at the 10 ns level and a spatial resolution of 6.96 μm. Aside from the intensity evolution of the damage spot, the corresponding quantized phase information could also be observed accurately by SUM–CDI, which is crucial to investigate the mechanism of ultrafast laser-induced damage, including the generation, propagation, and confrontation of shock waves, something which is difficult for other techniques without the capability of phase imaging. In addition, the temporal resolution is restricted by the pulse duration of the probe pulse and the time interval between adjacent probes, leaving room for potential improvement in temporal resolution to a Tfps rate. In addition, being a form of CDI, the theoretical resolution limitation of SUM–CDI is
APPENDIX A: COMPARISON BETWEEN SUM–CDI AND MULTIPLEXED CMI
To show the necessity of using varying incident angles
Figure 8.(a)–(d) Amplitude and (e)–(h) phase of the specimen corresponding to different times
Figure 9.(a), (b) Diffraction patterns of multiplexed CMI and SUM–CDI, respectively. (c), (d) Distributions of light on focal planes of multiplexed CMI and SUM–CDI, respectively.
Figure 10.Reconstructed amplitude and phase. (a), (b) Four reconstructed amplitudes and four reconstructed phases of multiplexed CMI with diffraction patterns in Fig.
APPENDIX B: SEPARATION OF FOUR DIFFRACTION PATTERNS CORRESPONDING TO FOUR PROBE LASER PULSES
In the experiment in Fig.
Figure 11.(a) Diffraction patterns recorded by the detector at different times with an interval of 2 s and each pattern contains four subpatterns corresponding to four pulse probes. (b)–(e) Corresponding subpatterns extracted from (a) with SUM–CDI. The scale bar in (a) is applicable to (b)–(e).
APPENDIX C: RECONSTRUCTION USING MULTIPLEXED CMI ALGORITHM WITH USAF 1951
Experiments were also carried out to check the performance of a multiplexed CMI algorithm with the experimental setup in Fig.
Figure 12.Experiment results of USAF 1951 (a)–(e) amplitude and (f)–(j) phase test target without the Dammann grating. (a) and (f) Diffraction patterns recorded by the detector. (b) and (g) Focus spots reconstructed from (a) and (f), respectively. (c) and (h) Corresponding retrieved amplitudes and phase distributions. (d) and (i) Enlarged views of the red boxes. (e) and (j) 1D curves of the blue lines. The unit of the colorbar is in radians.
References
[1] A. Schropp, R. Hoppe, V. Meier, J. Patommel, F. Seiboth, Y. Ping, D. G. Hicks, M. A. Beckwith, G. W. Collins, A. Higginbotham, J. S. Wark, H. J. Lee, B. Nagler, E. C. Galtier, B. Arnold, U. Zastrau, J. B. Hastings, C. G. Schroer. Imaging shock waves in diamond with both high temporal and spatial resolution at an XFEL. Sci. Rep., 5, 11089(2015).
[2] H. Yang, J. Cheng, Z. Liu, Q. Liu, L. Zhao, J. Wang, M. Chen. Dynamic behavior modeling of laser-induced damage initiated by surface defects on KDP crystals under nanosecond laser irradiation. Sci. Rep., 10, 500(2020).
[3] D. Giovanni, M. Righetto, Q. Zhang, J. W. M. Lim, S. Ramesh, T. C. Sum. Origins of the long-range exciton diffusion in perovskite nanocrystal films: photon recycling vs exciton hopping. Light Sci. Appl., 10, 2(2021).
[4] L. Lazovsky, D. Cismas, G. Allan, D. Given. CCD sensor and camera for 100 Mfps burst frame rate image capture. Proc. SPIE, 5787, 184-190(2005).
[5] V. Tiwari, M. A. Sutton, S. R. McNeill. Assessment of high speed imaging systems for 2D and 3D deformation measurements: methodology development and validation. Exp. Mech., 47, 561-579(2007).
[6] R. Kodama, K. Okada, Y. Kato. Development of a two-dimensional space-resolved high speed sampling camera. Rev. Sci. Instrum., 70, 625-628(1999).
[7] Z. Li, R. Zgadzaj, X. Wang, Y. Y. Chang, M. C. Downer. Single-shot tomographic movies of evolving light-velocity objects. Nat. Commun., 5, 3085(2014).
[8] K. Nakagawa, A. Iwasaki, Y. Oishi, R. Horisaki, A. Tsukamoto, A. Nakamura, K. Hirosawa, H. Liao, T. Ushida, K. Goda, F. Kannari, I. Sakuma. Sequentially timed all-optical mapping photography (STAMP). Nat. Photonics, 8, 695-700(2014).
[9] Q.-Y. Yue, Z.-J. Cheng, L. Han, Y. Yang, C.-S. Guo. One-shot time-resolved holographic polarization microscopy for imaging laser-induced ultrafast phenomena. Opt. Express, 25, 14182-14191(2017).
[10] X. Wang, L. Yan, J. Si, S. Matsuo, H. Xu, X. Hou. High-frame-rate observation of single femtosecond laser pulse propagation in fused silica using an echelon and optical polarigraphy technique. Appl. Opt., 53, 8395-8399(2014).
[11] H. Hu, T. Liu, H. Zhai. Comparison of femtosecond laser ablation of aluminum in water and in air by time-resolved optical diagnosis. Opt. Express, 23, 628-635(2015).
[12] A. Barty, S. Boutet, M. J. Bogan, S. Hau-Riege, S. Marchesini, K. Sokolowski-Tinten, N. Stojanovic, R. Tobey, H. Ehrke, A. Cavalleri, S. Düsterer, M. Frank, S. Bajt, B. W. Woods, M. M. Seibert, J. Hajdu, R. Treusch, H. N. Chapman. Ultrafast single-shot diffraction imaging of nanoscale dynamics. Nat. Photonics, 2, 415-419(2008).
[13] J. Barolak, D. Goldberger, C. Durfee, D. Adams. Single-shot ptychographic imaging of femtosecond laser induced plasma dynamics. Frontiers in Optics/Laser Science, FM1C.2(2020).
[14] R. Kodama, P. A. Norreys, K. Mima, A. E. Dangor, R. G. Evans, H. Fujita, Y. Kitagawa, K. Krushelnick, T. Miyakoshi, N. Miyanaga, T. Norimatsu, S. J. Rose, T. Shozaki, K. Shigemori, A. Sunahara, M. Tampo, K. A. Tanaka, Y. Toyama, T. Yamanaka, M. Zepf. Fast heating of ultrahigh-density plasma as a step towards laser fusion ignition. Nature, 412, 798-802(2001).
[15] X. Zeng, X. L. Mao, R. Greif, R. E. Russo. Experimental investigation of ablation efficiency and plasma expansion during femtosecond and nanosecond laser ablation of silicon. Appl. Phys. A, 80, 237-241(2005).
[16] X. Zeng, X. Mao, S. B. Wen, R. Greif, R. E. Russo. Energy deposition and shock wave propagation during pulsed laser ablation in fused silica cavities. J. Phys. D, 37, 1132-1136(2004).
[17] Q. Wang, L. Jiang, J. Sun, C. Pan, W. Han, G. Wang, H. Zhang, C. P. Grigoropoulos, Y. Lu. Enhancing the expansion of a plasma shockwave by crater-induced laser refocusing in femtosecond laser ablation of fused silica. Photon. Res., 5, 488-493(2017).
[18] J. Liang, L. Zhu, L. V. Wang. Single-shot real-time femtosecond imaging of temporal focusing. Light Sci. Appl., 7, 42(2018).
[19] L. Gao, J. Liang, C. Li, L. V. Wang. Single-shot compressed ultrafast photography at one hundred billion frames per second. Nature, 516, 74-77(2014).
[20] D. Qi, S. Zhang, C. Yang, Y. He, F. Cao, J. Yao, P. Ding, L. Gao, T. Jia, J. Liang, Z. Sun, L. V. Wang. “Single-shot compressed ultrafast photography: a review. Adv. Photon., 2, 014003(2020).
[21] S. Takazawa, J. Kang, M. Abe, H. Uematsu, N. Ishiguro, Y. Takahashi. Demonstration of single-frame coherent X-ray diffraction imaging using triangular aperture: towards dynamic nanoimaging of extended objects. Opt. Express, 29, 14394-14402(2021).
[22] J. Miao, P. Charalambous, J. Kirz, D. Sayre. Extending the methodology of X-ray crystallography to allow imaging of micrometre-sized non-crystalline specimens. Nature, 400, 342-344(1999).
[23] T. Ishikawa, H. Aoyagi, T. Asaka, Y. Asano, N. Azumi, T. Bizen, H. Ego, K. Fukami, T. Fukui, Y. Furukawa, S. Goto, H. Hanaki, T. Hara, T. Hasegawa, T. Hatsui, A. Higashiya, T. Hirono, N. Hosoda, M. Ishii, T. Inagaki, Y. Inubushi, T. Itoga, Y. Joti, M. Kago, T. Kameshima, H. Kimura, Y. Kirihara, A. Kiyomichi, T. Kobayashi, C. Kondo, T. Kudo, H. Maesaka, X. M. Maréchal, T. Masuda, S. Matsubara, T. Matsumoto, T. Matsushita, S. Matsui, M. Nagasono, N. Nariyama, H. Ohashi, T. Ohata, T. Ohshima, S. Ono, Y. Otake, C. Saji, T. Sakurai, T. Sato, K. Sawada, T. Seike, K. Shirasawa, T. Sugimoto, S. Suzuki, S. Takahashi, H. Takebe, K. Takeshita, K. Tamasaku, H. Tanaka, R. Tanaka, T. Tanaka, T. Togashi, K. Togawa, A. Tokuhisa, H. Tomizawa, K. Tono, S. Wu, M. Yabashi, M. Yamaga, A. Yamashita, K. Yanagida, C. Zhang, T. Shintake, H. Kitamura, N. Kumagai. A compact X-ray free-electron laser emitting in the sub-ångström region. Nat. Photonics, 6, 540-544(2012).
[24] P. Emma, R. Akre, J. Arthur, R. Bionta, C. Bostedt, J. Bozek, A. Brachmann, P. Bucksbaum, R. Coffee, F.-J. Decker, Y. Ding, D. Dowell, S. Edstrom, A. Fisher, J. Frisch, S. Gilevich, J. Hastings, G. Hays, P. Hering, Z. Huang, R. Iverson, H. Loos, M. Messerschmidt, A. Miahnahri, S. Moeller, H.-D. Nuhn, G. Pile, D. Ratner, J. Rzepiela, D. Schultz, T. Smith, P. Stefan, H. Tompkins, J. Turner, J. Welch, W. White, J. Wu, G. Yocky, J. Galayda. First lasing and operation of an ångstrom-wavelength free-electron laser. Nat. Photonics, 4, 641-647(2010).
[25] R. W. Gerchberg, W. O. Saxton. A practical algorithm for the determination of phase from image and diffraction plane pictures. Optik, 35, 237-246(1972).
[26] J. R. Fienup. Phase retrieval algorithms: a comparison. Appl. Opt., 21, 2758-2769(1982).
[27] C. Hu, Z. Du, M. Chen, S. Yang, H. Chen. Single-shot ultrafast phase retrieval photography. Opt. Lett., 44, 4419-4422(2019).
[28] O. Wengrowicz, O. Peleg, B. Loevsky, B. K. Chen, G. I. Haham, O. Cohen. Experimental demonstration of time-resolved imaging by multiplexed ptychography. Opt. Express, 27, 24568-24577(2019).
[29] P. Thibault, A. Menzel. Reconstructing state mixtures from diffraction measurements. Nature, 494, 68-71(2013).
[30] D. J. Batey, D. Claus, J. M. Rodenburg. Information multiplexing in ptychography. Ultramicroscopy, 138, 13-21(2014).
[31] X. Dong, X. Pan, C. Liu, J. Zhu. Single shot multi-wavelength phase retrieval with coherent modulation imaging. Opt. Lett., 43, 1762-1765(2018).
[32] F. Zhang, B. Chen, G. R. Morrison, J. Vila-Comamala, M. Guizar-Sicairos, I. K. Robinson. Phase retrieval by coherent modulation imaging. Nat. Commun., 7, 13367(2016).
[33] X. Pan, S. P. Veetil, C. Liu, H. Tao, Y. Jiang, Q. Lin, X. Li, J. Zhu. On-shot laser beam diagnostics for high-power laser facility with phase modulation imaging. Laser Phys. Lett., 13, 055001(2016).
[34] S. G. Demos, R. A. Negres, R. N. Raman, A. M. Rubenchik, M. D. Feit. Material response during nanosecond laser induced breakdown inside of the exit surface of fused silica. Laser Photon. Rev., 7, 444-452(2013).
[35] S. G. Demos, R. A. Negres, R. N. Raman, M. D. Feit, K. R. Manes, A. M. Rubenchik. Relaxation dynamics of nanosecond laser superheated material in dielectrics. Optica, 2, 765-772(2015).
[36] M. Boueri, M. Baudelet, J. Yu, X. Mao, S. S. Mao, R. Russo. Applied surface science early stage expansion and time-resolved spectral emission of laser-induced plasma from polymer. Appl. Surf. Sci., 255, 9566-9571(2009).
[37] Q. Wang, L. Jiang, J. Sun, C. Pan, W. Han, G. Wang, F. Wang, K. Zhang, M. Li, Y. Lu. Structure-mediated excitation of air plasma and silicon plasma expansion in femtosecond laser pulses ablation. Research, 2018, 11(2018).
[38] H. Hu, X. Wang, H. Zhai, N. Zhang, P. Wang. Generation of multiple stress waves in silica glass in high fluence femtosecond laser ablation. Appl. Phys. Lett., 97, 061117(2010).
[39] H. Zhang, F. Zhang, X. Du, G. Dong, J. Qiu. Influence of laser-induced air breakdown on femtosecond laser ablation of aluminum. Opt. Express, 23, 1370-1376(2015).
[40] H. Hu, X. Wang, H. Zhai. High-fluence femtosecond laser ablation of silica glass: effects of laser-induced pressure. J. Phys. D, 44, 135202(2011).
[41] Z. S. Márton, P. Heszler, Á. Mechler, B. Hopp, Z. Kántor, Z. S. Bor. Time-resolved shock-wave photography above 193-nm excimer laser-ablated graphite surface. Appl. Phys. A, 69, S133-S136(1999).
[42] L. I. Sedov. Similarity and Dimensional Methods in Mechanics(1982).
[43] C. Chang, X. Pan, H. Tao, C. Liu, S. P. Veetil, J. Zhu. Single-shot ptychography with highly tilted illuminations. Opt. Express, 28, 28441-28451(2020).
[44] Y. Xu, X. Pan, C. Liu, J. Zhu. Single-shot phase reconstruction based on beam splitting encoding and averaging. Opt. Express, 29, 43985-43999(2021).
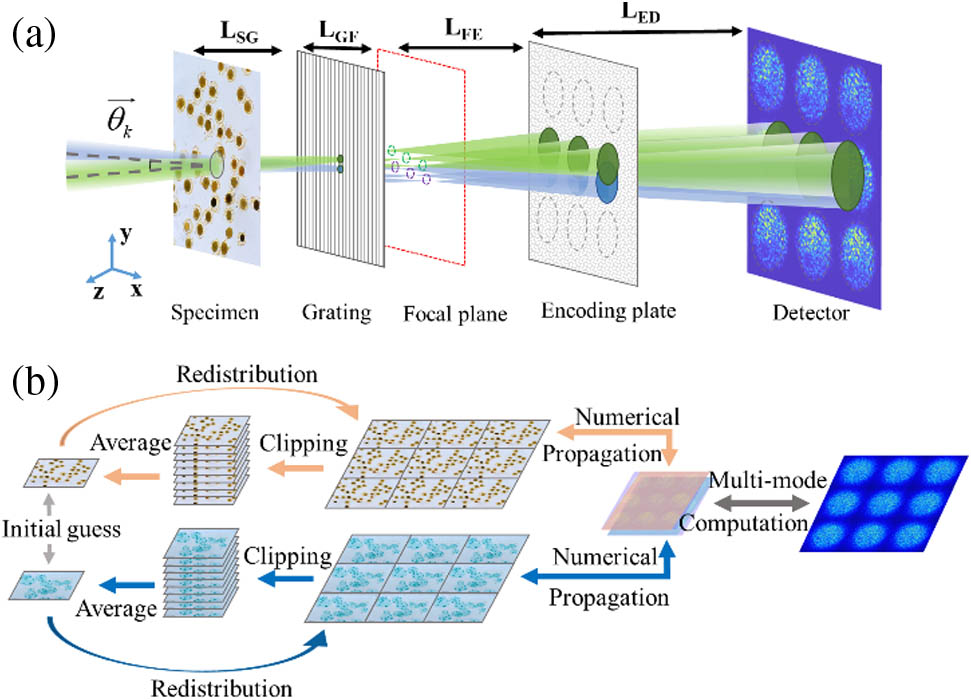
Set citation alerts for the article
Please enter your email address