Peter J. Winzer*
Abstract
This Review focuses on optical transmission fibers and the high-capacity systems operating thereon. It attempts to combine key lessons learned from the 50-year history of low-loss optical fibers with views on future fiber and systems requirements, discussing likely evolution paths and potential pitfalls in resolving the optical network capacity crunch.Human creativity, expressed in an endless stream of innovative digital applications and services, has fueled an enormous growth of fixed and mobile network traffic, sustained consistently over decades[1,2]. This growth has been uniquely enabled by fiber-optic transmission systems that have provided the economically viable network scalability based on which a truly global Internet could evolve, accessible by almost half of the world’s population today[3]. Recently, however, increasingly apparent scaling disparities[2] between technologies responsible for the generation and processing of information and technologies responsible for the transmission of information have led to severe optical network scalability problems, collectively referred to as the optical networks capacity crunch[4], which, if left unresolved, will hinder the further growth of digital services. This Review attempts to combine key lessons from the 50-year success story of optical fiber communications celebrated in this special issue of Chinese Optics Letters with visions for the evolution of future fiber optic networks, with the goal of pointing at important systems aspects that have historically impacted optical fiber designs and that are likely to impact new fiber designs in the future.
Until the seminal 1966 paper by Kao and Hockham[5], optical fibers and fiber bundles were thought to be usable only for short-range imaging and light delivery applications that could tolerate the high losses of then-existing optical glasses, on the order of 1000 dB/km[6]. Kao’s 1966 vision of ultra-low-loss optical fibers, which established the field of optical fiber communications, became a reality within just a few years[7], with fiber losses dwindling to below 1 dB/km, by more than 3 orders of magnitude, within a mere 6 years[8]; today’s lowest fiber loss is just below 0.15 dB/km[9].
The fiber-optic transmission systems of the 1970s and 1980s were single-span systems[6,10], with opto-electronic signal regeneration on the order of every 10 km. To increase repeater spacings as much as possible, the quest for reducing fiber losses and thereby moving transmission wavelengths to lower-loss windows went hand in hand with the quest for high-sensitivity opto-electronic transponders. This made coherent detection an attractive technology[11], widely studied and almost commercialized in the 1980s, despite severe problems associated with (then analog) local oscillator laser frequency and phase locking. The advent of the erbium-doped fiber amplifier[12,13] in the late 1980s, however, changed the situation completely. Low-noise optical amplification enabled long-haul transmissions of optical signals up to trans-Pacific distances without opto-electronic regeneration, and their broad amplification bandwidth made efficient wavelength-division multiplexing (WDM) possible. However, the optical amplifier also gave rise to a new set of transmission impairments due to signal distortions from fiber Kerr nonlinearities[14]. This opened up the search for new fibers, both with reduced (but nonzero) dispersions and larger effective core areas, combined with new system architectures using dispersion management to mitigate the impact of fiber nonlinearities[15]. The sum of all these techniques enabled unprecedented WDM capacity growth in the late 1990s, with commercial systems doubling their capacities each year for about 5 years during Internet boom times[1].
Sign up for Chinese Optics Letters TOC. Get the latest issue of Chinese Optics Letters delivered right to you!Sign up now
Importantly, from the mid-1970s to this day, fibers with improved loss and nonlinearity have continuously been deployed to enable higher-capacity transmission systems, while negligible amounts of installed fibers have been taken out of service. Figure 1 shows the cumulative amount of globally installed fiber kilometers[16], with over 3 billion km installed today, at a consistent rate of 30% per year pre-2000 and 15% per year post-2000. In 2016 alone, over 400 million fiber kilometers were installed, a significant fraction of which were associated with mobile network build-outs in China[16].
![Installed optical fiber worldwide (data: R. Mack, CRU International[16]).](/Images/icon/loading.gif)
Figure 1.Installed optical fiber worldwide (data: R. Mack, CRU International[16]).
Owing to further advances in fiber-optic transponders, which since have made ample use of digital electronic signal processing (DSP) with advanced optical modulation, coherent detection (now with digital phase locking), and coding[17], and despite continuing efforts to reduce fiber loss and nonlinearity, record systems experiments are starting to closely approach the fiber’s Shannon limit, cf., Fig. 2, depicting spectral efficiency (SE) versus transmission distance. (More precisely, the red line represents numerical estimates to lower bounds of fiber-dependent and network-configuration-dependent Shannon limits[18]).
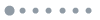
Figure 2.Shannon limit and record experimental SEs on available optical fibers.
Regarding further improvements in transmission fibers, it is clear from simple Shannon calculations that a reduction in loss or nonlinearity will not allow us to resolve the capacity problem. This is because both quantities only linearly improve the received signal-to-noise ratio (SNR), which has merely a logarithmic impact on the SE, , where the approximation holds for , valid at the high SEs of relevance. Therefore, a mere doubling of the SE requires a squaring of the received SNR, or a doubling of its dB value. Staring with a typical required SNR of 25 dB, this would imply fibers with a 0.0005 dB/km loss coefficient or a nonlinearity coefficient supporting a 25 dB higher signal power at the same level of nonlinear distortions. Both targets are practically, if not fundamentally, unrealistic. As an important conclusion from these observations, we note that low-loss, low-nonlinearity fibers, such as hollow-core fibers[19], will not be able to resolve the capacity crunch[2,20].
One possible way to significantly scale the capacity is to increase the transmission bandwidth. Figure 3 shows typical loss coefficients across the low-loss window of commercial optical fibers with (red) and without (blue) the characteristic OH absorption peak. In principle, a factor of in bandwidth could be gained () compared to the C-band (), where commercial transmission systems typically operate. However, it is very unlikely that this factor actually translates into a similar capacity gain; for example, fibers have somewhat higher losses outside the C-band, optical amplifiers have higher noise figures, band splitters in multi-band systems add further loss, and Raman pumps sharing spectrum with signal wavelengths will lead to yet hardly investigated problems[21]. In addition, practical concerns related to the maximum power that may be launched into a deployed optical fiber without invoking fiber fuse come into consideration[22]. In addition, as ultra-wideband systems involve different component technologies across the band, they will cost more per bit than single-band (C-band) systems. The only real benefit of ultra-wideband systems is their re-use of the vast, already installed fiber base. Deploying new fiber is very expensive, and prices vary widely depending on the deployment scenario, with per km assuming available duct space being a realistic assumption; deploying a 1,000-km cable () costs more than the WDM system operating over it. This basic commercial argument calls into question proposals for entirely new (e.g., photonic crystal) fiber deployments operating in the wide-band at nontelecom wavelengths. Not only would such systems be incompatible with existing systems and fiber spans (and hence not able to leverage the installed base), they would also require new component and subsystem technologies outside mature and high-volume C-band and possibly L-band solutions.
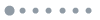
Figure 3.Low-loss window of commercial optical transmission fiber across telecommunication bands. Also shown are Raman pumps amplifying the L-band, which share spectra with S-band signals.
The above arguments against deploying radically new ultra-wide-band fibers might sound counter to what happened in the late 1970s, when fibers started to replace widely deployed twisted copper pairs, coaxial cables, and microwave relay towers for long-haul transport. To better understand this situation, Table 1 summarizes key system technologies of AT&T’s Long Lines business in the mid-1970s, with microwave relays (based on a massive network of relay towers) and coaxial cables responsible for 70% and 30% of long-haul traffic, respectively. Regarding coaxial cables, massive L-4 cables with a 3-inch outer diameter were exhausting duct space on many routes. These cables contained 10 coaxial pairs, could only be deployed in 400-m sections, offered repeater spacings in the 3-km range, and provided a total of 36,000 two-way voice channels[23]. In 1976 Bell Labs’ Atlanta Fiber Systems Experiment and the subsequent 1977 Chicago Project[24] demonstrated the first commercial (multi-mode) fiber system FT3, with 44.7 Mb/s per fiber and up to 144 fibers per ½-inch diameter cable, resulting in a potential cable capacity of over 50,000 two-way voice channels at a much increased regeneration distance of 6.5 km (cf. Table 1). Additional key advantages included the easier deployment of an order of magnitude thinner and two orders of magnitude lighter fiber-optic cables in longer sections and with a tighter bend radius, as well as at a significantly lower cost[6,10,25], which, together with other factors, such as moisture resistance and inherent electro-magnetic compatibility[6], quickly led to the adoption of fiber optics in long-haul transmissions as a replacement for all previously used and all other contemporarily contending technologies, such as millimeter-wave hollow waveguides[26]. By 1986, single-mode field demonstrations of the FTG system were matching previous per-cable capacities on a per-fiber basis, with over an order of magnitude increased repeater spacings compared to coaxial cables[27]. The deployment of WDM systems since the mid-1990s[28] immensely increased economically available network capacities, with today’s commercial systems carrying up to 70 Tb/s over a single optical fiber using both C- and L-bands. These orders of magnitude benefits, not just related to capacity but also to a host of other relevant deployment parameters, made the case for a radical infrastructure replacement, retiring proven technologies in favor of fibers. In today’s terms, in order for a new waveguide to be as revolutionary as fiber was over copper (and to therefore possibly warrant a complete infrastructure re-build), that waveguide would have to: immediately support 10 Pb/s; be scalable to 100 Eb/s in the future; allow for a repeater spacing of 2500 km (without any active elements between the transmitter and receiver); and yield a 10-fold reduction in cable diameter and weight compared to fiber. Clearly, such a waveguide is not even theoretically in sight, but these relative specifications once again underline the enormous achievements that optical fiber communications represents.
System | Year | Repeater Dist. [km] | Equiv. Capacity [Gb/s] | Cable Diam. [cm] | Cable Weight [kg/m] |
---|
Coaxial cable (L-4) | 1967 | 3 | 4.6 (per cable) | 7.6 | 14 |
Coaxial cable (L-5) | 1974 | 1.5 | 13.8 (per cable) | 7.6 | 14 |
Microw. relay (TD-2, TH-1) | 1969 | 40 | 1.9 (per link) | - | - |
Multi-mode fiber (FT3) | 1977 | 6.5 | 6.4 (per cable) | 1.3 | 0.25 |
Single-mode fiber (FTG) | 1986 | 23 | 3.4 (per fiber) | - | - |
Coherent PDM-WDM (Nokia 1830 PSS-2S) | 2016 | 100a (3000b) | ∼70,000 (per fiber) | - | - |
Table 1. Comparison of Long-Haul Transmission Technologies
As was the case with micro-processors around 2005, when clock frequencies could no longer scale to increase computational power, and parallelism in the form of multi-core processors had to be introduced, spatially parallel systems will have to be used to further scale optical network capacities. There is simply no other physical degree of freedom left to be exploited[29]. Notably, space division multiplexing (SDM) is almost as old as fiber itself, with both fiber and systems papers of already talking about the use of parallel fibers[6,30] to increase capacity. However, in its trivial form, where multiple transmission systems are deployed in parallel, SDM does not yield much advantage in terms of cost or energy per bit. The only cost reduction there would come from initial efficiency gains due to higher production volumes, but these would likely be insufficient to support sustained economic capacity growth. As with multi-core processors, integration will be key for future SDM systems[31]. Integration will take place across multiple system elements, including transponders, optical amplifiers, optical switches, and fibers, e.g., multi-core fibers (MCFs) or few-mode fibers (FMFs). SDM fibers supporting parallel spatial paths will eventually be more cost effective than individual strands of single-mode fibers; that this can be true is evidenced by multi-mode fibers, which support parallel spatial paths (modes) but are only slightly more expensive than single-mode fibers. SDM transponder arrays have a lower cost potential through -fold laser savings[32], and more energy-efficient submarine amplifiers are enabled by SDM[33].
Following the same arguments we made above for ultra-wideband systems, SDM fibers should be based on standard telecom wavelength bands to support a smooth upgrade from the vast base of already installed fibers[31]. As deployed cables start to be exhausted on certain routes, new cables will have to be added. These may contain bundles of conventional single-mode fibers or new SDM fibers (e.g., MCFs or FMFs) that should seamlessly integrate into the installed base. It is key for a successful comercialization of SDM technologies that they are able to operate on a hybrid infrastructure[34], just as today’s fiber-optic transmission systems can operate over a hybrid combination of different fiber types along a link. Deviating from this upgradability requirement may only be justifiable for systems that are deployed together with cables, such as submarine or data-center-related systems. These could, in principle, use SDM technologies that are incompatible with existing fibers and systems. However, overall subsystem cost considerations would quickly drive even these applications back to mature high-volume wavelength regions.
From the very beginnings of fiber optics, interfacing aspects were key considerations for commercial deployments, as stated in 1970 by Li and Marcatili[6]: “After we learn to make long, low-loss fibers, the single most difficult problem related to transmission may turn out to be the splicing of fibers in the field.” Another key aspect was the availability of reliable light sources, which had a strong impact on the type of fiber that was being deployed. For example, the reasons why multi-mode fiber was initially deployed included its relaxed connection tolerances and its natural interfacing to multi-mode light-emitting diodes[6,10]. The importance of efficient interfacing is equally valid today, e.g., for short-reach optical transmission, where low-cost multi-mode vertical cavity surface-emitting lasers dictate the use of more expensive multi-mode fibers. In future SDM systems, fiber interfacing and splicing will also play a significant role in SDM fiber choice.
Integrated SDM solutions, and in particular SDM fiber, may exhibit integration-induced crosstalk among their parallel spatial paths. In terms of its use in optical transmission systems, SDM fiber falls into two basic categories: fiber whose parallel propagating paths are sufficiently uncoupled to allow the detection of each path individually (the target design of MCF[35]), and fiber with non-negligible crosstalk between its spatial paths (e.g., FMF[36,37] and coupled-core MCF[38]). Importantly, the distinction of whether parallel spatial paths are “coupled” or “uncoupled” is a property of the system operating on the respective fiber, which intimately couples SDM fiber and system design. The tolerance of a system to crosstalk depends on the underlying modulation format[39]. SDM fiber that exceeds the allowed crosstalk (considering the total crosstalk from all interfering spatial paths, end-to-end, including connectors and splices) cannot be used unless all spatial paths are simultaneously received and digitally co-processed by multiple-input-multiple-output (MIMO) DSP to cancel multi-path crosstalk.
To assess how much crosstalk per unit length a nominally “uncoupled” MCF may allow between its cores, we first note that crosstalk scales linearly with the fiber length in weakly coupled MCFs[35], leading to the curves of Fig. 4, together with the shaded regions for realistic high-speed high-speed quadrature amplitude modulation (QAM)[17]. The curves show the modulation dependent maximum transmission distance as a function of MCF crosstalk per 1-km fiber segment. To the left of the respective lines, MIMO-DSP is needed; to the right, crosstalk can be treated as a perturbation with acceptable penalty. We next note that different transmission distances inherently permit different modulation formats. As per Fig. 2, the slope of this Shannon limit estimate as well as of the experimental records is around bit/s/Hz for each doubling in transmission distance. Conversely, an increase in SE by 4 bit/s/Hz (polarization-multiplexed), representing the steps from quadrature phase shift keying (QPSK) to 16-QAM to 64-QAM to 256-QAM, asks for successive reductions in reach by factors of . This is visualized by the markers in Fig. 4, which assume a QPSK baseline system reach of 10,000 km and scale the reach of higher-order QAM from there, both for ideal constellations (circles) and for realistic implementation penalties (squares). Importantly, the resulting allowable MCF crosstalk per unit length is nearly constant, around , irrespective of the underlying transmission system. Such high crosstalk requirements for MCF are very challenging end-to-end, including splices and fan-in/fan-out connectors[35]. The situation is even worse for MCF supporting multiple spatial modes per core, where crosstalk requirements are the same as for single-mode cores[40] but low crosstalk between multi-mode cores is harder to achieve[34]. These considerations call into question the feasibility of “uncoupled” MCF designs that do not limit high-performance systems by core-to-core crosstalk.
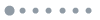
Figure 4.Maximum possible transmission distance vs. allowed crosstalk per km of MCF for various modulation formats. Owing to the inherent reach reduction with increasing QAM order, a universal MCF crosstalk specification of around emerges.
Interestingly, the possibility of propagating individual signals along differently angled rays over graded-index multimode fiber is as old as low-loss fiber itself[30], and various attempts have been made over the decades to exploit multi-path transmissions on multi-mode fibers, mostly in the form of mode-group multiplexing with offset launch and incoherent MIMO processing to mitigate modal crosstalk[41–43]. These experiments had moderate success, owing to their nonorthogonal mode launch and incoherent processing. The key to successful mode multiplexing over long-haul distances is the orthogonal excitation and coherent detection of the complete mode basis, with MIMO processing to invert unavoidable mode coupling upon transmission[44]. This is essentially the -dimensional extension of polarization-multiplexed coherent transmission. With the help of mode-selective orthogonal couplers (the -dimensional extension of polarization beam splitters), long-haul transmission has been demonstrated on FMF[36,37] and coupled-core MCF[37], the latter exhibiting homogenously strong coupling among all parallel transmission paths, which results in only a square-root growth of the differential modal group delay with fiber length and in a better nonlinear transmission performance[38].
Regarding the MIMO-DSP complexity, we note that the MIMO (polarization demultiplexing) part of today’s digital coherent ASICs occupies of the ASIC’s resources. Expanding this part to MIMO (10 spatial modes) results in a ASIC complexity increase[45], which is likely to be supported by future CMOS generations.
Coupled-mode transmission is not only interesting for the scaling of transmission capacity but may also enable new applications, such as information-theoretically secure transmission. As tapping into an SDM fiber by known means will result in severe mode-dependent loss (MDL), the presence of an eavesdropper can be readily detected at the legitimate receiver. At the same time, MDL experienced by an eavesdropper severely degrades its MIMO channel, making tapping into SDM fiber much harder compared to single-mode fiber (if not fundamentally impossible)[46]. In closing the circle to the imaging applications of fiber and fiber bundles prior to Kao’s 1966 vision of low-loss telecommunication fibers, it may well be that the DSP techniques studied today for MIMO-SDM transmission might find applications in other areas, such as imaging endoscopy through multi-mode fibers[47].
References
[1] R. W. Tkach. Bell Labs. Tech. J., 14, 3(2010).
[2] P. J. Winzer, D. T. Neilson. J. Lightwave Technol.(2017).
[3]
[4] A. R. Chraplyvy. European Conference on Optical Communication(2009).
[5] K. C. Kao, G. A. Hockham. Proc. IEE, 113, 1151(1966).
[6] T. Li, E. A. J. Marcatili. Bell Labs. Record, 331(1971).
[7] F. P. Kapron, D. B. Keck, R. D. Maurer. Appl. Phys. Lett., 17, 423(1970).
[8] M. Horiguchi, H. Osanai. El. Lett., 12, 310(1976).
[9] Y. Yamamoto, Y. Kawaguchi, M. Hirano. J. Lightwave Technol., 34, 321(2016).
[10] T. G. Giallorenzi. Proc. IEEE, 66, 744(1978).
[11] R. Linke, A. H. Gnauck. J. Lightwave Technol., 6, 1750(1988).
[12] R. J. Mears, L. Reekie, I. M. Jauncey, D. N. Payne. El. Lett., 23, 1026(1987).
[13] E. Desurvire, J. Simpson, P. C. Becker. Opt. Lett., 12, 888(1987).
[14] A. R. Chraplyvy. J. Lightwave Technol., 8, 1548(1990).
[15] R. W. Tkach, A. R. Chraplyvy, F. Forghieri, A. H. Gnauck, R. M. Derosier. J. Lightwave Technol., 13, 841(1995).
[16] R. Mack. CRU International.
[17] P. J. Winzer. J. Lightwave Technol., 30, 3824(2012).
[18] R.-J. Essiambre, G. Foschini, G. Kramer, P. J. Winzer, B. Goebel. J. Lightwave Technol., 28, 662(2010).
[19] P. J. Roberts, F. Couny, H. Sabert, B. J. Mangan, D. P. Williams, L. Farr, M. W. Mason, A. Tomlinson, T. A. Birks, J. C. Knight, P. S. J. Russell. Opt. Express, 13, 236(2005).
[20] R.-J. Essiambre, R. W. Tkach. Proc. IEEE, 100, 1035(2012).
[21] A. H. Gnauck, R. M. Jopson, P. J. Winzer. Photon. Technol. Lett.(2017).
[22] T. Morioka. Proceedings of OECC 2009, FT4(2009).
[23] G. H. Duval, L. M. Rackson. Bell Sys. Tech. J., 48, 1065(1969).
[24] M. I. Schwartz, W. A. Reenstra, J. H. Mullins, J. S. Cook. Bell Sys. Tech. J., 57, 1881(1969).
[25] M. E. Porter. Cases in Competitive Strategy(1983).
[26] W. D. Warters. Bell Sys. Tech. J., 56, 1825(1977).
[27] D. A. Fishman, S. Lumish, N. M. Denkin, R. R. Schulz, S. Y. Chai, K. Ogawa. Proceedings of OFC, PD11(1986).
[28] H. Kogelnik. Proceedings of ECOC, Mo1.1.1(2004).
[29] P. J. Winzer. IEEE/LEOS Newsletter, 2, 4(2009).
[30] T. Uchida, M. Furukawa, I. Kitano, K. Koizumi, H. Matsumura. IEEE J. Quantum. Electron., 6, 606(1970).
[31] P. J. Winzer. Nat. Photon., 8, 345(2014).
[32] P. J. Winzer. Bell Labs Tech. J., 19, 22(2014).
[33] H. Zhang, A. Turukhin, O. V. Sinkin, W. Patterson, H. G. Batshon, Y. Sun, C. R. Davidson, M. Mazurczyk, G. Mohs, D. Foursa, A. Pilipetskii. J. Lightwave Technol., 34, 1859(2016).
[34] N. K. Fontaine, T. Haramaty, R. Ryf, H. Chen, L. Miron, L. Pascar, M. Blau, B. Frenkel, L. Wang, Y. Messaddeq, S. LaRochelle, R.-J. Essiambre, Y.-M. Jung, Q. Kang, J. K. Sahu, S. Ul Alam, D. J. Richardson, D. M. Marom. Proceedings of OFC, Th5C.5(2015).
[35] K. Saitoh, S. Matsuo. J. Lightwave Technol., 34, 55(2016).
[36] E. Ip, M.-J. Li, K. Bennett, Y.-K. Huang, A. Tanaka, A. Korolev, K. Koreshkov, W. Wood, E. Mateo, J. Hu, Y. Yano. Proceedings of OFC, PDP5A.2(2013).
[37] R. Ryf, S. Randel, N. K. Fontaine, X. Palou, E. Burrows, S. Corteselli, S. Chandrasekhar, A. H. Gnauck, C. Xie, R.-J. Essiambre, P. J. Winzer, R. Delbue, P. Pupalaikis, A. Sureka, Y. Sun, L. Gruner-Nielsen, R. V. Jensen, R. Lingle. Proceedings of ECOC, We.2.D.1(2013).
[38] R. Ryf, J. C. Alvarado, B. Huang, J. A. Lopez, S. H. Chang, N. K. Fontaine, H. Chen, R.-J. Essiambre, E. Burrows, R. A. Correa, T. Hayashi, Y. Tamura, T. Hasegawa, T. Taru. Proceedings of ECOC, Th.3.C.3(2016).
[39] P. J. Winzer, A. H. Gnauck, A. Konczykowska, F. Jorge, J. Y. Dupuy. Proceedings of ECOC, Tu.5.B.7(2011).
[40] S. Randel, A. Sierra, R. Ryf, P. J. Winzer. Proceedings of ECOC, P4.08(2012).
[41] S. Berdague, P. Facq. Appl. Opt., 21, 1950(1982).
[42] H. R. Stuart. Science, 289, 281(2000).
[43] S. Schoellmann, N. Schrammar, W. Rosenkranz. Proceedings of OFC, JWA68(2008).
[44] P. J. Winzer, G. J. Foschini. Proceedings of OFC, OThO5(2011).
[45] S. Randel, P. J. Winzer, M. Monoliu, R. Ryf. Proceedings of ECOC, Th.2.C.4(2013).
[46] K. Guan, A. Tulino, P. J. Winzer, E. Soljanin. Trans. Inf. Forens. Secur., 10, 1325(2015).
[47] R. Y. Gu, R. N. Mahalati, J. M. Kahn. Opt. Express, 23, 26905(2015).