
- Photonics Research
- Vol. 9, Issue 5, 749 (2021)
Abstract
1. INTRODUCTION
Silicon photonics is a fascinating technology to realize large-scale electronics-photonics integration on a chip with low cost, high bandwidth, large volume, high energy efficiency, and complementary metal-oxide semiconductor (CMOS) compatibility [1–5]. Silicon photonics has been proving its great worth in data centers, long-haul telecommunication, integrated quantum communication, integrated microwave photonics, artificial intelligence (AI), and high-performance computers [2,4–8].
One of the key building blocks of silicon photonics is photodetectors (PDs) that convert high-speed optical signals to electrical signals [9,10]. However, the intrinsic properties of silicon (Si), an indirect band-gap semiconductor with a band-gap energy of 1.1 eV which is transparent in the near-IR wavelength band (1.3–1.55 μm), make it challenging to realize photodetection [10]. As an option, germanium (Ge), which possesses great linear absorption up to 1550 nm and can be extended up to 2000 nm by exploiting tensile-strained germanium-on-silicon (Ge-on-Si) bandgap shrinkage, has appeared as a prime choice for photodetection [11–14]. The measured absorption coefficients of Ge material are about
Figure 1.Three optical coupling schemes of Ge-on-Si PD: (a) vertical incidence and waveguide-integrated coupling including (b) butt coupling and (c) evanescent coupling. In evanescent coupling the optical input waveguide can be positioned on top, at the bottom, or lateral to the absorber (germanium). The inside of the orange rectangle is the evanescent-coupling configuration based on double lateral
Compared to a Si waveguide, the
Sign up for Photonics Research TOC. Get the latest issue of Photonics Research delivered right to you!Sign up now
In this paper, we report the first concept of a Ge-on-Si PD with double lateral
We design and fabricate the Ge-on-Si PD with double lateral coupling
2. STRUCTURE AND PRINCIPLE
Figure 2.(a) Three-dimensional (3D) schematic of Ge-on-Si PD with double lateral
For regular butt-coupling and evanescent-coupling (bottom) schemes, the light is injected into Ge region all at once with nonuniform optical field distribution in the absorber, which will cause a large electron–hole pair density at the Ge-Si waveguide interface [13,25]. The high density of photogenerated electron–hole pairs generates relatively strong gradient of charge, which will induce a large electric field opposing to the applied external voltage. This phenomenon is called carrier screening [39,40], which will drastically degrade the 3 dB OE bandwidth. Therefore, the uniform optical field distribution in the Ge region will be helpful to generate homogeneous electron–hole pair density, and consequently, it is beneficial to improving the operation speed. Figure 2(d) shows the cross-sectional view of field distribution of the
3. EXPERIMENTAL RESULTS
A. Optical Micrograph and Setups
Figure 3.(a) Micrograph of the fabricated Ge-on-Si PD with double lateral
B. Static Measurements
Figure 4.(a) Current-voltage (
C. Equivalent Circuit Model
Figure 5.(a) and (b) Experimental and fitted magnitude/phase part of the small-signal S11 reflection parameters from 100 MHz to 60 GHz at
D. Theoretical 3 dB OE Bandwidth
To profoundly analyze the high-frequency response of the fabricated Ge-on-Si PD with double lateral
The total 3 dB frequency response, determined by
E. Small-Signal Measurements
Figure 6.(a) Normalized RF response of the Ge-on-Si PD with lateral
F. Large-Signal Eye Diagram Measurements
Figure 7.Measured 70, 80, 90, and 100 Gbit/s NRZ eye diagrams under 3 V reverse-bias voltage.
Figure 8.Measured 100, 120, 140, and 150 Gbit/s PAM-4 eye diagrams under 3 V reverse-bias voltage.
Figure 9.Measured 60 Gbit/s NRZ eye diagrams with 5, 10, 15, and 20 mA photocurrent at the DC bias voltage of
4. DISCUSSION
Compared to the butt-coupling scheme, the possible freely chosen parameters of an evanescent-coupling configuration based on double lateral
In our proposed structure, the silicon-based
5. CONCLUSION
In summary, as a proof-of-concept demonstration, we have proposed a novel light coupling scheme of waveguide-integrated Ge-on-Si PD: lateral coupling by employing double lateral
References
[1] R. A. Soref. Silicon-based optoelectronics. Proc. IEEE, 81, 1687-1706(1993).
[2] D. A. B. Miller. Device requirements for optical interconnects to silicon chips. Proc. IEEE, 97, 1166-1185(2009).
[3] M. Asghari, A. V. Krishnamoorthy. Energy-efficient communication. Nat. Photonics, 5, 268-270(2011).
[4] D. Thomson, A. Zilkie, J. E. Bowers, T. Komljenovic, G. T. Reed, L. Vivien, D. Marris-Morini, E. Cassan, L. Virot, J. M. Fédéli, J.-M. Hartmann, J. H. Schmid, D. X. Xu, F. Boeuf, P. O’Brien, G. Z. Mashanovich, M. Nedeljkovic. Roadmap on silicon photonics. J. Opt., 18, 073003(2016).
[5] K. Yamada, T. Tsuchizawa, H. Nishi, R. Kou, T. Hiraki, K. Takeda, H. Fukuda, Y. Ishikawa, K. Wada, T. Yamamoto. High-performance silicon photonics technology for telecommunications applications. Sci. Technol. Adv. Mater., 15, 024603(2014).
[6] G. Zhang, J. Y. Haw, H. Cai, F. Xu, S. M. Assad, J. F. Fitzsimons, X. Zhou, Y. Zhang, S. Yu, J. Wu, W. Ser, L. C. Kwek, A. Q. Liu. An integrated silicon photonic chip platform for continuous-variable quantum key distribution. Nat. Photonics, 13, 839-842(2019).
[7] Y. Shen, N. Harris, S. Skirlo, M. Prabhu, T. Baehr-Jones, M. Hochberg, X. Sun, S. Zhao, H. Larochelle, D. Englund, M. Soljačić. Deep learning with coherent nanophotonic circuits. Nat. Photonics, 11, 441-446(2017).
[8] D. Marpaung, J. Yao, J. Capmany. Integrated microwave photonics. Nat. Photonics, 13, 80-90(2019).
[9] D. Marris-Morini, V. Vakarin, J. M. Ramirez, Q. Liu, A. Ballabio, J. Frigerio, M. Montesinos, C. Alonso-Ramos, X. Le Roux, S. Serna, D. Benedikovic, D. Chrastina, L. Vivien, G. Isella. Germanium based integrated photonics from near- to mid-infrared applications. Nanophotonics, 7, 1781-1793(2018).
[10] J. Michel, J. Liu, L. C. Kimerling. High-performance Ge-on-Si photodetectors. Nat. Photonics, 4, 527-534(2010).
[11] Y. Ishikawa, K. Wada, D. D. Cannan, J. Liu, D. L. Hsin-Chiao, L. C. Kimerling. Strain-induced band gap shrinkage in Ge grown on Si substrate. Appl. Phys. Lett., 82, 2044-2046(2003).
[12] J. F. Liu, J. Michel, W. Giziewicz, D. Pan, K. Wada, D. Cannon, L. C. Kimerling, J. Chen, F. O. Ilday, F. X. Kartner, J. Yasaitis. High-performance, tensile strained Ge p-i-n photodetectors on a Si platform. Appl. Phys. Lett., 87, 103501(2005).
[13] R. Anthony, D. E. Hagan, D. Genuth-Okon, L. M. Maestro, I. F. Crowe, M. P. Halsall, A. P. Knights. Extended wavelength responsivity of a germanium photodetector integrated with a silicon waveguide exploiting the indirect transition. IEEE J. Sel. Top. Quantum Electron., 26, 3800107(2020).
[14] H. Chen, M. Galili, P. Verheyen, P. De Heyn, G. Lepage, J. De Coster, S. Balakrishnan, P. Absil, L. Oxenlowe, J. Van Campenhout, G. Roelken. 100-Gbps RZ data reception in 67-GHz Si-contacted germanium waveguide p-i-n photodetectors. J. Lightwave Technol., 35, 722-726(2017).
[15] M. Rouviere, M. Halbwax, J.-L. Cercus, E. Cassan, L. Vivien, D. Pascal, M. Heitzmann, J.-M. Hartmann, S. Laval. Integration of germanium waveguide photodetectors for intrachip optical interconnects. Opt. Eng., 44, 75402-75406(2005).
[16] D. Dai, M. Piels, J. E. Bowers. Monolithic germanium/silicon photodetectors with decoupled structures: resonant APDs and UTC photodiodes. IEEE J. Sel. Top. Quantum Electron., 20, 3802214(2014).
[17] L. Colace, G. Masini, F. Galluzzi, G. Assanto, G. Capellini, L. Di Gaspare, E. Palange, F. Evangelisti. Metal-semiconductor-metal near-infrared light detector based on epitaxial Ge/Si. Appl. Phys. Lett., 72, 3175-3177(1998).
[18] H. C. Luan, D. R. Lim, K. K. Lee, K. M. Chen, L. C. Kimerling. High-quality Ge epilayers on Si with low threading-dislocation densities. Appl. Phys. Lett., 75, 2909-2911(1999).
[19] M. Halbwax, D. Bouchier, V. Yam, D. Debarre, L. H. Nguyen, Y. Zheng, P. Rosner, M. Benamara, H. P. Strunk, C. Clerc. Kinetics of Ge growth at low temperature on Si (001) by ultrahigh vacuum chemical vapor deposition. J. Appl. Phys., 97, 064907(2005).
[20] J. Liu, R. Camacho-Aguilera, J. T. Bessette, X. Sun, X. Wang, Y. Cai, L. C. Kimerling, J. Michel. Ge-on-Si optoelectronics. Thin Solid Films, 520, 3354-3360(2012).
[21] L. Chen, P. Dong, M. Lipson. High performance germanium photodetectors integrated on submicron silicon waveguides by low temperature wafer bonding. Opt. Express, 16, 11513-11518(2008).
[22] Z. Huang, J. Oh, J. C. Campbell. Back-side-illuminated high-speed Ge photodetector fabricated on Si substrate using thin SiGe buffer layers. Appl. Phys. Lett., 85, 3286-3288(2004).
[23] D. Ahn, C. Hong, J. Liu, W. Giziewicz, M. Beals, L. C. Kimerling, J. Michel. High performance, waveguide integrated Ge photodetectors. Opt. Express, 15, 3916-3921(2007).
[24] X. Li, L. Peng, Z. Liu, X. Liu, J. Zheng, Y. Zuo, C. Xue, B. Cheng. High-power back-to-back dual-absorption germanium photodetector. Opt. Lett., 45, 1358-1361(2020).
[25] H. Chen, P. Verheyen, P. De Heyn, G. Lepage, J. De Coster, P. Absil, G. Roelkens, J. Van Campenhout. High-responsivity low-voltage 28-Gb/s Ge p-i-n photodetector with silicon contacts. J. Lightwave Technol., 33, 820-824(2015).
[26] H. Chen, P. Verheyen, P. De Heyn, G. Lepage, J. De Coster, S. Balakrishnan, P. Absil, W. Yao, L. Shen, G. Roelkens, J. Van Campenhou. −1 V bias 67 GHz bandwidth Si-contacted germanium waveguide p-i-n photodetector for optical links at 56 Gbps and beyond. Opt. Express, 24, 4622-4631(2016).
[27] Z. Liu, F. Yang, W. Wu, H. Cong, J. Zheng, C. Li, C. Xue, B. Cheng, Q. Wang. 48 GHz high-performance Ge-on-SOI photodetector with zero-bias 40 Gbps grown by selective epitaxial growth. J. Lightwave Technol., 35, 5306-5310(2017).
[28] D. Benedikovic, L. Virot, G. Aubin, F. Amar, B. Szelag, B. Karakus, J. M. Hartmann, C. Alonso-Ramos, X. L. Roux, P. Crozat, E. Cassan, D. Marris-Morini, C. Baudot, F. Boeuf, J. M. Fédéli, C. Kopp, L. Vivien. 25 Gbps low-voltage hetero-structured silicon-germanium waveguide pin photodetectors for monolithic on-chip nanophotonic architectures. Photon. Res., 7, 437-444(2019).
[29] D. Benedikovic, L. Virot, G. Aubin, J. M. Hartmann, F. Amar, B. Szelag, X. L. Roux, C. Alonso-Ramos, P. Crozat, É. Cassan, D. Marris-Morini, C. Baudot, F. Boeuf, J. M. Fédéli, C. Kopp, L. Vivien. Comprehensive study on chip-integrated germanium PIN photodetectors for energy-efficient silicon interconnects. IEEE J. Sel. Top. Quantum Electron., 56, 8400409(2020).
[30] L. Vivien, M. Rouvière, J. M. Fédéli, D. Marris-Morini, J. F. Damlencourt, J. Mangeney, P. Crozat, L. E. Melhaoui, E. Cassan, X. L. Roux, D. Pascal, S. Laval. High speed and high responsivity germanium photodetector integrated in a silicon-on-insulator microwaveguide. Opt. Express, 15, 9843-9848(2007).
[31] C. T. DeRose, D. C. Trotter, W. A. Zortman, A. L. Starbuck, M. Fisher, M. R. Watts, P. S. Davids. Ultra compact 45 GHz CMOS compatible germanium waveguide photodiode with low dark current. Opt. Express, 19, 24897-24904(2011).
[32] J. Cui, Z. Zhou. High-performance Ge-on-Si photodetector with optimized DBR location. Opt. Lett., 42, 5141-5144(2017).
[33] D. Ahn, L. C. Kimerling, J. Michel. Efficient evanescent wave coupling conditions for waveguide-integrated thin-film Si/Ge photodetectors on silicon-on-insulator/germanium-on-insulator substrates. J. Appl. Phys., 110, 083115(2011).
[34] M. J. Byrd, E. Timurdogan, Z. Su, C. V. Poulton, M. R. Watts. Mode-evolution-based coupler for high saturation power Ge-on-Si photodetectors. Opt. Lett., 42, 851-854(2017).
[35] C. H. Henry, R. F. Kazarinov, H. J. Lee, K. J. Orlowsky, L. E. Katz. Low loss Si3N4-SiO2 optical waveguides on Si. Appl. Opt., 26, 2621-2624(1987).
[36] P. Muñoz, G. Micó, L. A. Bru, D. Pastor, D. Pérez, J. D. Doménech, J. Fernández, R. Baños, B. Gargallo, R. Alemany, A. M. Sánchez, J. M. Cirera, R. Mas, C. Domínguez. Silicon nitride photonic integration platforms for visible, near-infrared and mid-infrared applications. Sensors, 17, 2088(2017).
[37] C. G. H. Roeloffzen, M. Hoekman, E. J. Klein, L. S. Wevers, R. B. Timens, D. Marchenko, D. Geskus, R. Dekker, A. Alippi, R. Grootjans, A. V. Rees, R. M. Oldenbeuving, J. P. Epping, R. G. Heideman, K. Worhoff, A. Leinse, D. Geuzebroek, E. Schreuder, P. W. L. van Dijk, I. Visscher, C. Taddei, Y. Fan, C. Taballione, Y. Liu, D. Marpaung, L. Zhuang, M. Benelajla, K. J. Boller. Low-loss Si3N4 TriPleX optical waveguides: technology and applications overview. IEEE J. Sel. Top. Quantum Electron., 24, 4400321(2018).
[38] D. J. Blumenthal, R. Heideman, D. Geuzebroek, A. Leinse, C. Roeloffzen. Silicon nitride in silicon photonics. Proc. IEEE, 106, 2209-2231(2018).
[39] K. S. Giboney, M. J. W. Rodwell, J. E. Bowers. Travelling-wave photodetector design and measurements. IEEE J. Sel. Top. Quantum Electron., 2, 622-629(1996).
[40] A. Beling, X. Xie, J. C. Campbell. High-power, high-linearity photodiodes. Optica, 3, 328-338(2016).
[41] M. Oehme, J. Werner, E. Kasper, M. Jutzi, M. Berroth. High bandwidth Ge p-i-n photodetector integrated on Si. Appl. Phys. Lett., 89, 071117(2006).
[42] X. Hu, D. Wu, H. Zhang, W. Li, D. Chen, L. Wang, X. Xiao, S. Yu. High-speed lateral PIN germanium photodetector with 4-directional light input. Opt. Express, 28, 38343-38354(2020).
[43] Y. Zuo, Y. Yu, Y. Zhang, D. Zhou, X. L. Zhang. Integrated high-power germanium photodetectors assisted by light field manipulation. Opt. Lett., 44, 3338-3341(2019).
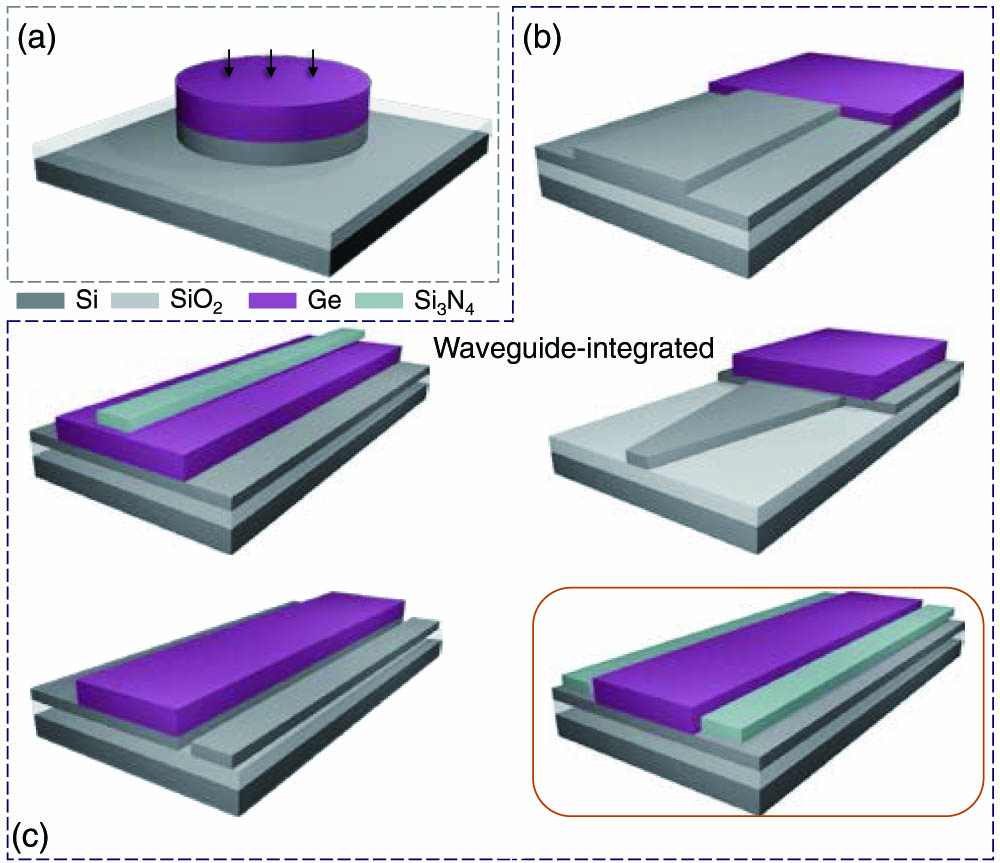
Set citation alerts for the article
Please enter your email address