
- Photonics Research
- Vol. 10, Issue 3, 711 (2022)
Abstract
1. INTRODUCTION
First observed in bulk materials by Chiao, Townes, and Stoicheff in 1964 [1], stimulated Brillouin scattering (SBS) is a nonlinear optical process in which light is backscattered by a hypersonic acoustic wave. The Doppler-shifted backward signal beats with the pump light, creating a moving interference pattern that in turn amplifies the acoustic wave, leading to strong amplification of the backward signal for high enough pump power. Since its first demonstration, SBS has been explored and exploited in many different systems, especially optical fibers [2] and integrated photonics [3]. Among the many applications of SBS are narrow linewidth lasers [4], fiber sensors [5], light storage systems [6], and microwave photonic filters [7]. To date, to the best of our knowledge, SBS has not been studied in chiral photonic crystal fibers (PCFs), where the core microstructure rotates with the position along the fiber axis.
Studies of SBS in nonchiral PCFs with high air-filling fractions and very small cores have revealed how tight confinement of acoustic vibrations gives rise to SBS frequency shifts not seen in standard step-index fibers [8,9]. In recent years, chiral PCF, drawn from a spinning preform, has emerged as a unique platform to study the behavior of light in chiral structures that are infinitely extended in the direction of the twist [10]. Such structures are very difficult, if not impossible, to realize on an integrated photonic chip. Chiral PCF has been shown to robustly preserve a circular polarization state over long distances, allowing the investigation of nonlinear processes in the presence of chirality [11,12]. Although the polarization properties of SBS in nonchiral fibers have been investigated by many research groups [13–16], it has so far, to the best of our knowledge, not been possible to observe SBS between clean circularly polarized modes.
Here, we report the first experimental study of SBS in chiral PCF, demonstrating both Brillouin amplification of circularly polarized light, and a continuous-wave (CW) circularly polarized Brillouin laser. The results are of potential interest in fiber-optic current sensing [17], fiber-optic gyroscopes [18], and teleportation of quantum states [19].
Sign up for Photonics Research TOC. Get the latest issue of Photonics Research delivered right to you!Sign up now
2. CHIRAL PHOTONIC CRYSTAL FIBER
Figure 1(a) shows a 3D sketch of a chiral PCF. Figure 1(b) shows a scanning electron microscopy (SEM) image of the microstructure of the chiral PCF used in the experiments. The chiral PCF was designed to support only a nondegenerate pair of circularly polarized fundamental modes. It was fabricated from fused silica using the standard stack-and-draw technique. The helical pitch was 1.6 cm, created by spinning the preform during the fiber draw, and the circular birefringence
Figure 1.(a) 3D sketch of a chiral PCF. (b) SEM image of the PCF used in the experiments. The hollow channels have a diameter
3. BRILLOUIN SCATTERING IN CHIRAL PCF
Figure 2(a) shows the heterodyne detection setup for measuring Brillouin frequency in a chiral PCF. Both pump and local oscillator (LO) are derived from a narrow linewidth (
Figure 2.(a) Experimental setup to measure Brillouin frequencies in a chiral PCF. (b) Spontaneous Brillouin spectrum generated by pumping with 0.9 W of circularly polarized CW laser at 1550 nm. (c) Numerically calculated optoacoustic coupling coefficients (normalized) for different acoustic modes around 11 GHz. (d) Axial displacements (normalized to the square root of the power) of acoustic modes at 11.021 GHz and 11.039 GHz in (c).
To confirm these results, we used FEM to calculate the normalized optoacoustic coupling coefficient, defined by [21]
We next increased the pump power to reach the SBS regime, when the polarization state of the much stronger Stokes signals could be more easily and precisely measured (Fig. 3). The CW pump light was amplified in an EDFA and its polarization state was controlled using a combination of polarizing beamsplitter (PBS) and quarter-wave (
Figure 3.(a) Experimental setup to measure SBS threshold of different circularly polarized light in chiral PCF. EDFA, erbium-doped fiber amplifier; FPC, fiber-based polarization controller; and PBS, polarizing beamsplitter. (b) Stokes and transmitted pump power in a 38 m length of chiral PCF for LCP, RCP, and linearly polarized pump light (left to right).
These results confirm robust maintenance of circular polarization states and conservation of spin during Brillouin scattering in chiral PCF. More interestingly, when a linearly polarized pump is injected into the chiral PCF, the polarization state of the backscattered Stokes wave is also linearly polarized and has the same azimuthal angle as that of the pump, as shown in far right figure in Fig. 3(b). We attribute this to well-controlled optical activity in the chiral PCF, which causes the linearly polarized pump and backward Stokes modes to be co-polarized at all points along the fiber. (For more details, see Appendix D.) The SBS threshold power in all the three cases was
To assess the robustness of polarization maintenance, the Stokes signal powers and Stokes polarization states were measured for LCP and RCP pump light with the fiber spooled to diameters of 50 and 16 cm (Table 1). The pump power was kept constant at 2.45 W in all the measurements. The modulus of the Stokes parameter
Signal Powers and Stokes Parameter
LCP Stokes (mW) | RCP Stokes (mW) | Trans. Pump (mW) | ||||||
---|---|---|---|---|---|---|---|---|
LCP pump | 44 | 3 | 720 | 693 | 628 | 632 | 0.885 | 0.991 |
RCP pump | 618 | 667 | 38 | 4 | 641 | 652 | 0.884 | 0.988 |
Pump power is 2.45 W. SD, spool diameter (in cm).
To illustrate the striking contrast between chiral and nonchiral PCFs, we carried out the same experiment using a 25 m long untwisted PCF with a closely similar microstructure. The fiber loss was 0.012 dB/m and its slightly two-fold rotationally symmetric structure [Fig. 1(b)] resulted in a linear birefringence
Figure 4.Stokes signal powers measured in a 25 m length of untwisted PCF, pumped by LCP, RCP, and linearly polarized light.
4. BRILLOUIN AMPLIFICATION IN CHIRAL PCF
We now investigate Brillouin amplification of circularly polarized light using a 2 m length of chiral PCF. The amplified Stokes signal takes the well-known form
Figure 5.(a) Measured Brillouin gain spectra (FWHM 41 MHz) when pumping with LCP (left) and RCP (right) light. The circles are measured data, and the full red line is a fit based on two Lorentzians (FWHM 31 and 65 MHz), indicated by the dashed red lines. (b) Left: Stokes gain spectra for LCP pump powers 0.54 W, 0.86 W, 1.36 W, and 2.09 W. Right: peak Stokes gain as a function of LCP pump power. The circles are the experimental data points and the line is a linear fit.
The peak gain coefficients of the two Lorentzians are
5. CIRCULARLY POLARIZED BRILLOUIN LASER
Next, we constructed a circularly polarized Brillouin laser by placing a 2 m length of chiral PCF in a ring cavity [Fig. 6(a)]. A CW pump light was launched into the laser cavity through a polarizing beamsplitter and a
Figure 6.(a) Experimental setup of circularly polarized Brillouin laser. (b) Laser power as a function of pump power for LCP (blue circles) and RCP (red crosses) pump light. (c) Theoretically fitted (blue) and measured (red) laser spectrum from sub-coherence delayed self-heterodyne system. Four sharp side peaks around the center peak is from the artifacts of the pump laser and unrelated to the experimental result. The inset is the spectrum of spontaneously scattered Stokes light from the identical chiral PCF and the linewidth-narrowed intracavity laser spectrum measured by delayed self-heterodyne system (the axis labels are same as those of the main figure).
The spectrum measured by the OSA just before the filter confirmed the presence of a lasing signal with frequency 11.013 GHz below the pump frequency. (For details, see Appendix E.) The laser power is plotted against the LCP and RCP pump power in Fig. 6(b). Lasing commences when the Brillouin gain exceeds the 4.12 dB round-trip loss of the laser cavity (beamsplitter 1 dB; fiber coupling 1.5 dB; fiber loss 0.12 dB; polarization components 1.5 dB). The threshold powers are
We note that lasers with a sub-kHz linewidth have a coherence length of greater than 100 km, and the decoherent self-heterodyning requires the SMF in reference path to be longer than that. Such a long fiber transmission will induce Gaussian noise on the laser spectrum. To precisely measure the Brillouin laser linewidth, we applied a “sub-coherence” self-heterodyne technique using a 2 km length of single-mode fiber as a delay line, so that the path difference was less than the laser coherence length and the laser spectrum has less Gaussian noise. The exact laser linewidth of 660 Hz is then obtained by fitting the measured spectrum to the sub-coherence lineshape function [Fig. 6(c)].
6. CONCLUSIONS
Chiral PCF, drawn from a spinning preform, robustly maintains the spin of guided light and therefore may be used for stimulated Brillouin scattering with circularly polarized light. Conservation of the angular momentum means that significant gain is only possible when the Stokes and pump signals are orthogonally polarized. Brillouin amplifiers and lasers for circularly polarized light can be successfully realized using a chiral PCF as the gain medium. When, in contrast, a nonchiral PCF is used, the polarization states of the Stokes and transmitted pump signals are unpredictable.
To the best of our knowledge, this is the first report of SBS in circularly birefringent chiral fiber. We note that chiral fibers with off-center [23] or coupled cores [24] can also display circular birefringence, although fibers with two-fold rotational symmetry, such as twisted polarization-maintaining fiber (PMF) [25], support elliptically polarized eigenmodes. Twisted multicore PCFs with
Acknowledgment
Acknowledgment. The authors thank Yang Chen, Jennifer Hartwigs, and Jiapeng Huang for help with several aspects of theory and experiments.
APPENDIX A: SIMULATIONS OF OPTICAL AND ACOUSTIC MODES
The finite element method (FEM) simulations were based on an SEM image of the fiber structure. To increase the accuracy, the mesh density was increased within the core area. The hollow channel diameter
Figure 7.(a) Calculated normalized electric fields and effective indices for LCP and RCP modes. (b) Calculated axial displacements and frequencies for two acoustic modes that contribute to the backward Brillouin scattering in the chiral PCF.
Figure
APPENDIX B: ESTIMATE OF BRILLOUIN GAIN
We can roughly estimate the gain coefficient using [
APPENDIX C: ANGULAR MOMENTUM CONSERVATION
The forward-propagating pump mode in a chiral PCF takes the analytical form,
APPENDIX D: POLARIZATION MAINTENANCE FOR LINEARLY POLARIZED PUMP
When the pump light is linearly polarized, the circular birefringence of the chiral PCF causes optical activity (i.e., the direction of polarization rotates by
APPENDIX E: MEASUREMENT OF BRILLOUIN AMPLIFICATION, GAIN, AND BRILLOUIN LASER SPECTRUM
The Brillouin gain and spectrum were measured using a typical pump-seed setup with added polarization control, as shown in Fig.
Figure 8.Experimental setup to measure Brillouin gain spectra of the chiral PCF. (Dashed line represents free space.)
Figure 9.Power spectrum of the laser output before the narrowband filter, measured by the OSA. The lasing and back-reflected pump signals are clearly observed.
APPENDIX F: DELAYED SELF-HETERODYNE SETUP FOR LINEWIDTH MEASUREMENT
Since grating-based OSAs and Fabry–Perot interferometers typically have insufficient resolution (a few GHz and tens of MHz, respectively), we employed delayed interferometric self-heterodyning to measure the Brillouin laser linewidth. Based on a Mach–Zehnder interferometer, this system can measure sub-kHz linewidths. The setup is shown in Fig.
Figure 10.(a) Experimental setup for delayed self-heterodyne measurement. (b) Experimentally measured self-heterodyne spectrum when the SMF delay is 26 km. (c) Experimentally measured self-heterodyne spectrum (red) and fitted curves (blue) with sub-coherent heterodyne formula [31] when the SMF delay is 2 km and 3 km.
Analysis of the resulting spectrum falls into two regimes: one for lasers with a coherence length shorter than or comparable with the SMF delay, and the other for lasers with a coherence length longer than the SMF delay (the sub-coherence domain). Ideally, the laser with a coherence length shorter than the imbalance delay will produce a Lorentzian line with a half-width-at-half-maximum equal to the laser linewidth. Due, however, to substantial Gaussian noise (pump noise, vibrations, and acoustic noise) during long SMF transmission, the resulting spectrum is a convolution of Gaussian and Lorentzian functions and has a complex Voigt profile, as shown in Fig.
References
[1] R. Y. Chiao, C. H. Townes, B. P. Stoicheff. Stimulated Brillouin scattering and coherent generation of intense hypersonic waves. Phys. Rev. Lett., 12, 592-595(1964).
[2] A. Kobyakov, M. Sauer, D. Chowdhury. Stimulated Brillouin scattering in optical fibers. Adv. Opt. Photon., 2, 1-59(2010).
[3] B. J. Eggleton, C. G. Poulton, P. T. Rakich, M. J. Steel, G. Bahl. Brillouin integrated photonics. Nat. Photonics, 13, 664-677(2019).
[4] S. P. Smith, F. Zarinetchi, S. Ezekiel. Narrow-linewidth stimulated Brillouin fiber laser and applications. Opt. Lett., 16, 393-395(1991).
[5] X. Bao, L. Chen. Recent progress in Brillouin scattering based fiber sensors. Sensors, 11, 4152-4187(2011).
[6] B. Stiller, M. Merklein, C. Wolff, K. Vu, P. Ma, S. J. Madden, B. J. Eggleton. Coherently refreshing hypersonic phonons for light storage. Optica, 7, 492-497(2020).
[7] A. Zarifi, M. Merklein, Y. Liu, A. Choudhary, B. J. Eggleton, B. Corcoran. Wide-range optical carrier recovery via broadened Brillouin filters. Opt. Lett., 46, 166-169(2021).
[8] P. Dainese, P. St.J. Russell, N. Y. Joly, J. C. Knight, G. S. Wiederhecker, H. L. Fragnito, V. Laude, A. Khelif. Stimulated Brillouin scattering from multi-GHz-guided acoustic phonons in nanostructured photonic crystal fibres. Nat. Phys., 2, 388-392(2006).
[9] J. C. Beugnot, T. Sylvestre, D. Alasia, H. Maillotte, V. Laude, A. Monteville, L. Provino, N. Traynor, S. F. Mafang, L. Thevenaz. Complete experimental characterization of stimulated Brillouin scattering in photonic crystal fiber. Opt. Express, 15, 15517-15522(2007).
[10] P. St.J. Russell, R. Beravat, G. K. L. Wong. Helically twisted photonic crystal fibres. Phil. Trans. R. Soc. A, 375, 20150440(2017).
[11] R. P. Sopalla, G. K. L. Wong, N. Y. Joly, M. H. Frosz, X. Jiang, G. Ahmed, P. St.J. Russell. Generation of broadband circularly polarized supercontinuum light in twisted photonic crystal fibers. Opt. Lett., 44, 3964-3967(2019).
[12] S. Davtyan, D. Novoa, Y. Chen, M. H. Frosz, P. St.J. Russell. Polarization-tailored Raman frequency conversion in chiral gas-filled hollow-core photonic crystal fibers. Phys. Rev. Lett., 122, 143902(2019).
[13] M. O. van Deventer, A. J. Boot. Polarization properties of stimulated Brillouin scattering in single-mode fibers. J. Lightwave Technol., 12, 585-590(1994).
[14] D. Williams, X. Bao, L. Chen. Effects of polarization on stimulated Brillouin scattering in a birefringent optical fiber. Photon. Res., 2, 126-137(2014).
[15] K. Y. Song, W. Zou, Z. He, K. Hotate. All-optical dynamic grating generation based on Brillouin scattering in polarization-maintaining fiber. Opt. Lett., 33, 926-928(2008).
[16] G. Prabhakar, X. Liu, J. Demas, P. Gregg, S. Ramachandran. Phase conjugation in OAM fiber modes via stimulated Brillouin scattering. Conference on Lasers and Electro-Optics, FTh1M.4(2018).
[17] R. Beravat, G. K. L. Wong, X. M. Xi, M. H. Frosz, P. St.J. Russell. Current sensing using circularly birefringent twisted solid-core photonic crystal fiber. Opt. Lett., 41, 1672-1675(2016).
[18] V. M. N. Passaro, A. Cuccovillo, L. Vaiani, M. De Carlo, C. E. Campanella. Gyroscope technology and applications: a review in the industrial perspective. Sensors, 17, 2284(2017).
[19] K. Tsurumoto, R. Kuroiwa, H. Kano, Y. Sekiguchi, H. Kosaka. Quantum teleportation-based state transfer of photon polarization into a carbon spin in diamond. Commun. Phys., 2, 74(2019).
[20] R. W. Boyd. Nonlinear Optics(2008).
[21] V. Laude, A. Khelif, S. Benchbane, M. Wilm, T. Sylvestre, B. Kibler, A. Mussot, J. M. Dudley, H. Maillotte. Phononic band-gap guidance of acoustic modes in photonic crystal fibers. Phys. Rev. B, 71, 045107(2005).
[22] T. Okoshi, K. Kikuchi, A. Nakayama. Novel method for high resolution measurement of laser output spectrum. Electron. Lett., 16, 630-631(1980).
[23] R. D. Birch. Fabrication and characterisation of circularly birefringent helical fibres. Electron. Lett., 23, 50-52(1987).
[24] S. Loranger, Y. Chen, P. Roth, M. H. Frosz, G. K. L. Wong, P. St.J. Russell. Bragg reflection and conversion between helical Bloch modes in chiral three-core photonic crystal fiber. J. Lightwave Technol., 38, 4100-4107(2020).
[25] R. I. Laming, D. N. Payne. Electric-current sensors employing spun highly birefringent optical fibers. J. Lightwave Technol., 7, 2084-2094(1989).
[26] X. Zeng, W. He, J. Huang, P. Roth, M. H. Frosz, G. K. L. Wong, B. Stiller, P. St.J. Russell. Stimulated Brillouin scattering of helical Bloch modes in 3-fold rotationally symmetric chiral 4-core photonic crystal fibre. CLEO, CD-6.4(2021).
[27] A. Küng, P.-A. Nicati, P. A. Robert. Brillouin fiber optic current sensor. Optical Fiber Sensors, We21(1996).
[28] M. E. J. Friese, T. A. Nieminen, N. R. Heckenberg, H. Rubinsztein-Dunlop. Optical alignment and spinning of laser-trapped microscopic particles. Nature, 394, 348-350(1998).
[29] S. Huang, L. Thevenaz, K. Toyama, B. Y. Kim, H. J. Shaw. Optical Kerr-effect in fiber-optic Brillouin ring laser gyroscopes. IEEE Photon. Technol. Lett., 5, 365-367(1993).
[30] J. C. Knight, T. A. Birks, P. St.J. Russell, D. M. Atkin. All-silica single-mode optical fiber with photonic crystal cladding. Opt. Lett., 21, 1547-1549(1996).
[31] L. Richter, H. Mandelberg, M. Kruger, P. McGrath. Linewidth determination from self-heterodyne measurements with subcoherence delay times. IEEE J. Quantum Electron., 22, 2070-2074(1986).
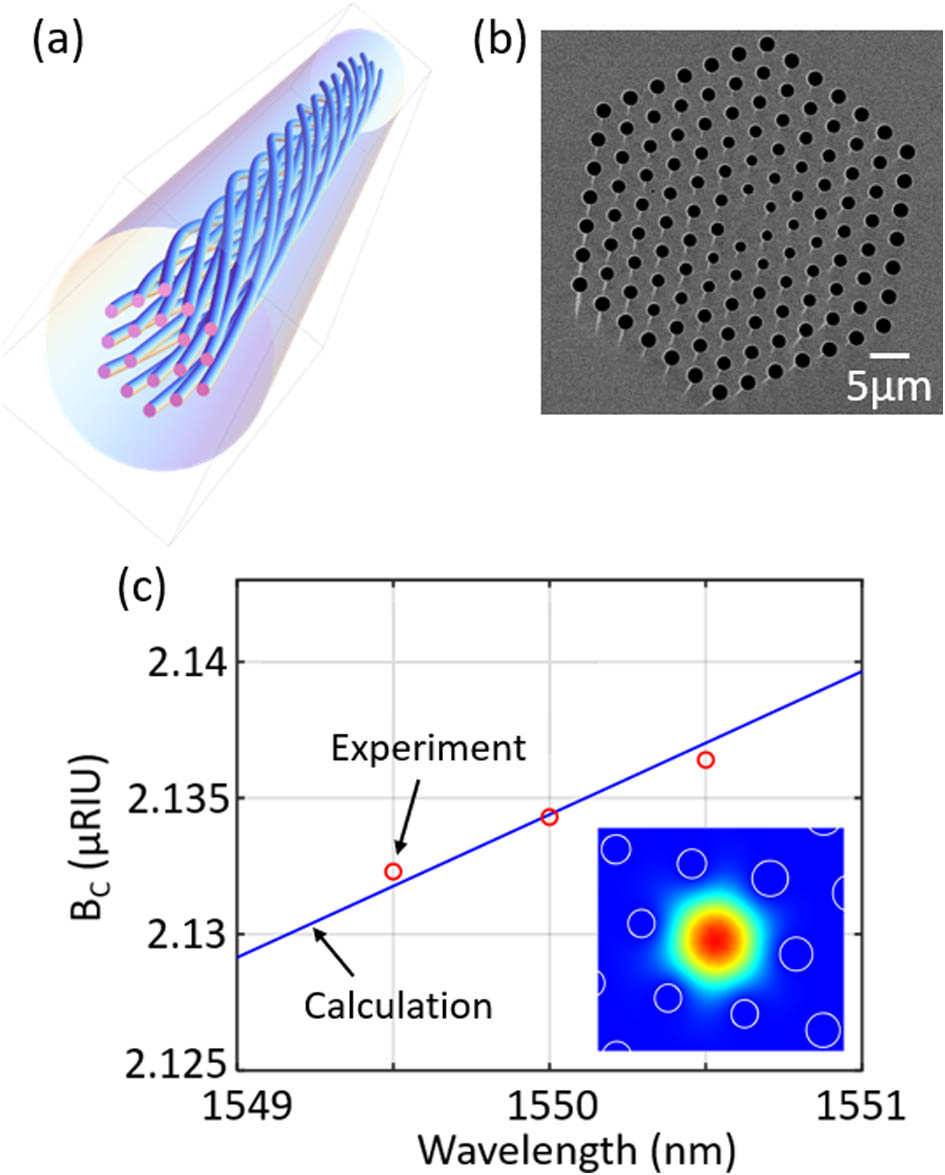
Set citation alerts for the article
Please enter your email address