
- Chinese Optics Letters
- Vol. 19, Issue 11, 111601 (2021)
Abstract
1. Introduction
New materials and technologies, such as metamaterials[
In this paper, ITO is selected to design a periodic helix structure. Each helix unit cell consists of four helices with different radii. The simulation results show that the structure has flat-topped broadband CD, which is unattainable for the Au or Ag helix structures.
2. Structure Design
A periodic helix array structure was designed in this paper. Each unit cell consists of four subunits, each of which is composed of a helix, and the different helices have different radii, as shown in Fig. 1. The helix radii of the four subunits are
Sign up for Chinese Optics Letters TOC. Get the latest issue of Chinese Optics Letters delivered right to you!Sign up now
Figure 1.Schematic diagrams of a chiral unit cell composed of four helices with different radii as subunits.
A finite-difference time-domain (FDTD) method software was employed to simulate chiral properties of the structure. Due to the periodicity of the structure, we therefore considered a single unit cell with periodic boundary conditions in both
3. Simulation Results and Discussion
By FDTD simulations, the transmission spectra of RCP and LCP waves were calculated and substituted into the CD formula below:
Figure 2.CD and transmittances of the structure for the RCP and LCP incident waves.
We also simulated the distributions of electric field intensities in different cross sections vertical to the incident direction for the RCP and LCP waves at the wavelength of 6.44 µm, which is shown in Fig. 3. The cross sections were selected at 0.2 µm intervals from the incident end to the exit end. It can be seen from the figure that the field intensity at the upper end of the structure for the RCP wave incidence is the strongest, the plasmon resonance in ITO is also the strongest, and the field intensity gradually decreases from top to bottom. However, for the LCP wave incidence, the field intensity as well as the plasmon resonance is the strongest in the middle of the structure, and the plasmon resonances at the two ends weaken. These fields occur mainly on the surfaces of the helices, indicating that surface plasmon polaritons (SPPs) are induced. The couplings of the SPPs between different helices also appear, inflencing the CD responses of structures. By comparing them, the plasmon resonance caused by the RCP wave is stronger than the one for the LCP wave incidence. Therefore, the structure absorbs the RCP wave more strongly than the LCP wave, resulting in an obvious CD response. This is the same as the results shown in Fig. 2.
Figure 3.Distributions of electric field intensities at different cross sections vertical to the incident direction under the RCP and LCP waves at the wavelength of 6.44 µm. (a) z = −1.2 µm; (b) z = −1.0 µm; (c) z = −0.8 µm; (d) z = −0.6 µm; (e) z =−0.4 µm; (f) z = −0.2 µm.
It is assumed that the structure is illuminated by a plane wave propagating in the positive
A complex Jones matrix
For circular states, the
The unit cell of the model proposed is composed of four subunits with different helix radii. In order to compare easily, four subunits were simulated separately, and the period remained the same as that of the original unit cell. Each subunit is in the middle of the unit cell. Their CD curves are shown in Fig. 4. The chirality of metamaterial structures usually occurs near the resonance frequencies of the structures. The resonance frequencies are closely related to the sizes of the structures. From this figure, it can be seen that for one of the subunits, corresponding resonance frequencies exist. With the increasing radius of the helix, the resonance of the subunit shifts towards the longer wavelengths. Their CD bandwidths are all narrower than that of the unit cell. The reason lies in that the unit cell is composed of four subunits with different helix sizes. Multiple resonance peaks caused by different subunits work together, leading to CD broadening.
Figure 4.CD curves of the original structure and single helix structures with radii of 0.30 µm, 0.35 µm, 0.40 µm, and 0.45 µm, respectively.
We change the number of turns of each subunit helix to keep them different. The simulated CD curves for two different cases are shown in Fig. 5, in which the original CD curve for the one turn helix is also plotted. Case one: the number of turns of the helix with the smallest radius is set to 1.07, the number of turns of the helix with the largest radius is set to 0.93, and those of the other two subunits remain at one. Case two: the number of turns of the helix with the second smallest radius is set to 1.04, and other parameters are the same as those of case one. It is found that for the adjusted structure, their CD values from 4.5 µm to 7 µm change between 0.265 and 0.274, while the CD values for the same number of turns change between 0.251 and 0.288. It means that the CD distributions with the adjusted structure are flatter, which provides a design idea for the flat-topped broadband CD.
Figure 5.CD properties of the structure with the different number of turns of subunits.
To investigate the influences of structural materials on CD responses, we change the component of helices and replace ITO with Au and Ag. The other parameters remain unchanged. The dielectric constants of Au and Ag come from experimental data[
Figure 6.CD properties of the original structure and structures with different components consisting of Ag and Au.
Optical activity refers to the rotation ability of a polarized wave passing through the chiral structure, which can be expressed as
Figure 7.Optical activity distributions of the structures with different components consisting of ITO, Ag, and Au, respectively.
4. Conclusion
In this paper, we proposed a periodic chiral structure, in which each unit cell consisting of four ITO helix subunits with different radii realized flat-topped broadband CD and optical activity in the mid-infrared band. The simulation results show that by optimizing parameters of the helix structure, flat-topped broadband CD distributions with their values from 4.5 µm to 7 µm can be obtained. The comparisons between the ITO helix structures and metallic (Ag and Au) helix structures show that the CD and the optical activity of the former have broader bands, which provides a new idea for the design of broadband polarization state control devices in the mid-infrared band. Future work will be to further research the mechanism of chirality and explore improvement to the design of broadband chiral devices.
References
[1] N. M. Estakhri, B. Edwards, N. Engheta. Inverse-designed metastructures that solve equations. Science, 363, 1333(2019).
[2] L. L. Spada, L. Vegni. Near-zero-index wires. Opt. Express, 25, 23699(2017).
[3] J. Y. Xiao, R. W. Xiao, R. X. Zhang, Z. X. Shen, W. Hu, L. Wang, Y. Q. Lu. Tunable terahertz absorber based on transparent and flexible metamaterial. Chin. Opt. Lett., 18, 092403(2020).
[4] N. J. Greybush, V. Pacheco-Peña, N. Engheta, C. B. Murray, C. R. Kagan. Plasmonic optical and chiroptical response of self-assembled Au nanorod equilateral trimers. ACS Nano, 13, 3875(2019).
[5] H. A. Atwater. The promise of plasmonics. Sci. Am., 296, 56(2007).
[6] F. Mattioli, G. Mazzeo, G. Longhi, S. Abbate, G. Pellegrini, E. Mogni, M. Celebrano, M. Finazzi, L. Duo, C. G. Zanchi, M. Tommasini, M. Pea, S. Cibella, R. Polito, F. Sciortino, L. Baldassarre, A. Nucara, M. Ortolani, P. Biagioni. Plasmonic superchiral lattice resonances in the mid-infrared. ACS Photon., 7, 2676(2020).
[7] I. H. Lee, D. Yoo, P. Avouris, T. Low, S. H. Oh. Graphene acoustic plasmon resonator for ultrasensitive infrared spectroscopy. Nat. Nanotechnol., 14, 313(2019).
[8] K. S. Novoselov, A. K. Geim, S. V. Morozov, D. Jiang, Y. Zhang, S. V. Dubonos, I. V. Grigorieva, A. A. Firsov. Electric field effect in atomically thin carbon films. Science, 306, 666(2004).
[9] J. H. Hu, J. Fu, X. H. Liu, D. P. Ren, J. J. Zhao, Y. Q. Huang. Perfect absorption in a monolayer graphene at the near-infrared using a compound waveguide grating by robust critical coupling. Chin. Opt. Lett., 17, 010501(2019).
[10] L. L. Spada, C. Spooner, S. Haq, Y. Hao. Curvilinear meta surfaces for surface wave manipulation. Sci. Rep., 9, 3107(2019).
[11] N. Meinzer, W. L. Barnes, I. R. Hooper. Plasmonic meta-atoms and metasurfaces. Nat. Photonics, 8, 889(2014).
[12] A. Kuzyk, R. Schreiber, Z. Y. Fan, G. Pardatscher, E.-M. Roller, A. Hogele, C. Friedrich, F. C. Simmel, A. O. Govorov, T. Liedl. DNA-based self-assembly of chiral plasmonic nanostructures with tailored optical response. Nature, 483, 311(2012).
[13] Z. Lalegani, S. A. S. Ebrahimi, B. Hamawandi, L. L. Spada, M. S. Topark. Modeling, design, and synthesis of gram-scale monodispersed silver nanoparticles using microwave-assisted polyol process for metamaterial applications. Opt. Mater., 108, 110381(2020).
[14] R. Knipper, V. Kopecký, U. Huebner, J. Popp, T. G. Mayerhöfer. Slit-enhanced chiral- and broadband infrared ultra-sensing. ACS Photon., 5, 3238(2018).
[15] K. Tanaka, D. Arslan, S. Fasold, M. Steinert, J. Sautter, M. Falkner, T. Pertsch, M. Decker, I. Staude. Chiral bilayer all-dielectric metasurfaces. ACS Nano, 14, 15926(2020).
[16] K. Höflich, T. Feichtner, E. Hansjürgen, C. Haverkamp, H. Kollmann, C. Lienau, M. Silies. Resonant behavior of a single plasmonic helix. Optica, 6, 1098(2019).
[17] C. He, T. Sun, J. J. Guo, M. Cao, J. Xia, J. P. Hu, Y. Yan, C. H. Wang. Metalens of circular polarization dichroism with helical surface arrays in mid-infrared region. Adv. Opt. Mater., 7, 1901129(2019).
[18] L. K. Khorashad, L. V. Besteiro, M. A. Correa-Duarte, S. Burger, Z. M. Wang, A. O. Govorov. Hot electrons generated in chiral plasmonic nanocrystals as a mechanism for surface photochemistry and chiral growth. J. Am. Chem. Soc., 142, 4193(2020).
[19] S. Droulias, L. Bougas. Surface plasmon platform for angle-resolved chiral sensing. ACS Photon., 6, 1485(2019).
[20] J. Kaschke, M. Blome, S. Burger, M. Wegener. Tapered N-helical metamaterials with three-fold rotational symmetryas improved circular polarizers. Opt. Express, 22, 19936(2014).
[21] J. Zhang, R. Tu, C. Huang, X. L. Yao, X. Hu, H. X. Ge, X. F. Zhang. Chiral plasmonic nanostructure of twistedly stacked nanogaps. Chin. Opt. Lett., 19, 013601(2021).
[22] A. V. Rogacheva, V. A. Fedotov, A. S. Schwanecke, N. I. Zheludev. Giant gyrotropy due to electromagnetic-field coupling in a bilayered chiral structure. Phy. Rev. Lett., 97, 177401(2006).
[23] S. Zhang, Y. S. Park, J. Li, X. Li, W. Zhang, X. Zhang. Negative refractive index in chiral metamaterials. Phys. Rev. Lett., 102, 023901(2009).
[24] Y. Fang, R. Verre, L. Shao, P. Nordlander, M. Käll. Hot electron generation and cathodoluminescence nanoscopy of chiral split ring resonators. Nano Lett., 16, 5183(2016).
[25] T. Fu, Y. Qu, T. Wang, G. Wang, Y. Wang, H. Li, J. Li, L. Wang, Z. Zhang. Tunable chiroptical response of chiral plasmonic nanostructures fabricated with chiral templates through oblique angle deposition. J. Phys. Chem. C, 121, 1299(2017).
[26] Y. Z. He, G. K. Larsen, W. Ingram, Y. P. Zhao. Tunable three-dimensional helically stacked plasmonic layers on nanosphere monolayers. Nano Lett., 14, 1976(2014).
[27] Y. Z. He, K. Lawrence, W. Ingram, Y. P. Zhao. Strong local chiroptical response in racemic patchy silver films: enabling a large-area chiroptical device. ACS Photon., 2, 1246(2015).
[28] R. Kolkowski, L. Petti, M. Rippa, C. Lafargue, J. Zyss. Octupolar plasmonic meta-molecules for nonlinear chiral watermarking at subwavelength scale. ACS Photon., 2, 899(2015).
[29] V. E. Bochenkov, D. S. Sutherland. Chiral plasmonic nanocrescents: large-area fabrication and optical properties. Opt. Express, 26, 27101(2018).
[30] A. G. Mark, J. G. Gibbs, T. C. Lee, P. Fischer. Hybrid nanocolloids with programmed three-dimensional shape and material composition. Nat. Mater., 12, 802(2013).
[31] C. R. Han, L. C. Yang, P. Ye, E. P. J. Parrott, E. Pickwell-Macpherson, W. Y. Tam. Three dimensional chiral plasmon rulers based on silver nanorod trimers. Opt. Express, 26, 10315(2018).
[32] E. S. A. Goerlitzer, R. Mohammadi, S. Nechayev, P. Banzer, N. Vogel. Large-area 3D plasmonic crescents with tunable chirality. Adv. Opt. Mater., 7, 1801770(2019).
[33] J. K. Gansel, M. Thiel, M. S. Rill, M. Decker, K. Bade, V. Saile, G. V. Freymann, S. Linden, M. Wegener. Gold helix photonic metamaterial as broadband circular polarizer. Science, 325, 1513(2009).
[34] M. Schnell, P. Sarriugarte, T. Neuman, A. B. Khanikaev, R. Hillenbrand. Real-space mapping of the chiral near-field distributions in spiral antennas and planar metasurfaces. Nano Lett., 16, 663(2016).
[35] S. J. Zhang, Y. Li, Z. P. Liu, J. L. Ren, Y. F. Xiao, H. Yang, Q. Gong. Two-photon polymerization of a three dimensional structure using beams with orbital angular momentum. Appl. Phy. Lett., 105, 061101(2014).
[36] Y. L. Zhu, B. W. Cao, J. W. Li, Y. Wu, A. X. Lu, L. Y. Qian, C. Q. Han, C. C. Yan. L-shaped ITO structures fabricated by oblique angle deposition technique for mid-infrared circular dichroism. Opt. Express, 27, 33243(2019).
[37] G. V. Naik, V. M. Shalaev, A. Boltasseva. Alternative plasmonic materials: beyond gold and silver. Adv. Mater., 25, 3264(2013).
[38] K. Gansel, M. Latzel, A. Frolich, J. Kaschke, M. Thiel, M. Wegener. Tapered gold-helix metamaterials as improved circular polarizers. Appl. Phy. Lett., 100, 101109(2012).
[39] K. Johannes, M. Wegener. Gold triple-helix mid-infrared metamaterial by STED-inspired laser lithography. Opt. Lett., 40, 3986(2015).
[40] E. D. Palik. Handbook of Optical Constants of Solids(1997).
[41] C. Menzel, C. Rockstuhl, F. Lederer. Advanced Jones calculus for the classification of periodic metamaterials. Phys. Rev. A, 82, 053811(2010).
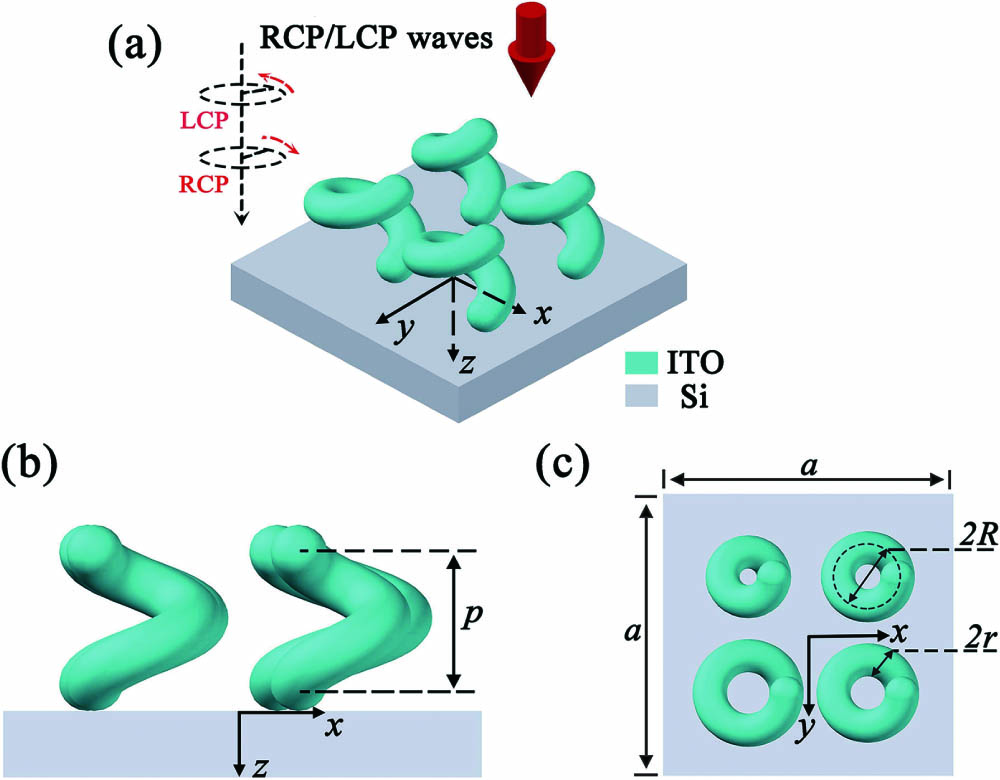
Set citation alerts for the article
Please enter your email address