
- Chinese Optics Letters
- Vol. 19, Issue 7, 071403 (2021)
Abstract
1. Introduction
It is well known that a light beam with a phase singularity nested in its wave front carries an orbital angular momentum (OAM) equivalent to
The complicated phase pattern of light beam carrying OAM is of particular interest in modern optics[
The SMCW structure can support various unique oscillating guided modes, i.e., the ultrahigh-order modes (UOMs). Critical coupling of a specific UOM can be achieved when the mode leakage interferes destructively with the reflected beam, resulting in a resonant dip in the reflection spectrum and an M-line in the scattered beams. The high sensitivity of the UOMs is very useful and has been applied in various fields, e.g., we have recently demonstrated an enhanced Imbert–Fedorov effect directly without quantum weak measurement[
Sign up for Chinese Optics Letters TOC. Get the latest issue of Chinese Optics Letters delivered right to you!Sign up now
Our experiments show that the reflected pattern varies significantly near the UOMs’ resonance, whilst several concentric rings are severely distorted as the twisted M-line sweeps across the light spot. The usual transfer matrix method for the waveguide fails on this issue since the beam axis of the VB cannot be applied to define the incident angle. Instead of applying Fourier analysis, which describes the light propagation using a plane-wave basis, we find that a simple model in the ray optics picture is sufficient. We replace the common incident angle by a local incident angle, which is defined via the energy flux over the whole cross section of the VB; then, the reflected pattern is simulated with high accuracy. Furthermore, the evolution of the M-line depends crucially on the phase structure and can be used for detection purposes. This work helps us to understand the mode coupling process using twisted photons and may provide an effective approach to the sensing of complicated phase structures.
2. Experimental Results
The schematic in Fig. 1 shows an incident VB reflected by an SMCW chip, and the insert shows the distribution of a specific UOM. The SMCW structure is similar to the metal–dielectric–metal (MDM) scheme that is widely studied in plasmonics. Instead of the basic symmetric and antisymmetric modes, our interest mainly focuses on the oscillating modes of high order. In our experiment, a 0.23 mm thick glass slab with excellent optical parallelism is sandwiched between two silver films. The excited UOMs will propagate along the millimeter thick glass layer until all of the energy is leaked out through the metal claddings. The upper coupling layer is made of 30 nm thick silver film, which facilitates the direct coupling into the chip from free space. No high index prism or coupling grating is needed, since the effective index of UOMs can approach zero. The metal substrate is also 30 nm thick, so both reflection and transmission can be detected. Theoretically, it is simple to calculate the reflectivity and field distribution of a Gaussian beam via the transfer matrix method. What we are more interested in is the reflection of an incident beam carrying OAM at the resonance of the UOMs.
Figure 1.Refraction of an optical VB by an SMCW chip and the E-field distribution of an oscillating UOM at resonance (red line).
A VB generated by a spiral phase plate is incident on the surface of the SMCW with an angle
Figure 2.(a) Optical field of the incident beam generated by a spiral phase plate with l = 1. (b), (c), and (d) Optical field of the reflected beam at 9.42, 9.50, and 9.52 deg, respectively. The intensity is normalized to the maximum of the incident light spot.
Figure 3.(Top) Experimentally measured reflectivity of a VB reflected by the SMCW chip; (bottom) numerically simulated reflectivity of a planar wave model via the transfer matrix method. The permittivity of silver is based on the Drude model εAg = −13.9235 + 0.7233i, and the permittivity of the guiding layer is 2.25.
The inner ring is formed by the direct reflection of incidence at the chip surface. The physics behind the occurrence of outer rings can be attributed to the random scattering caused by the natural roughness of the silver film, which enables the coupling between different UOMs of different orders in all azimuthal directions[
In Fig. 3, the measured reflectivity of a VB is compared with the simulated results. Since the number of the UOMs in the sub-millimeter thick SMCW is very large, we only show a part of the spectrum for clarity. The FWHM of the dips in the experiment is much wider, which can be attributed to the different incidence conditions and will be explained later. In Fig. 4, we show the reflection of an ordinary Gaussian beam near resonance, which differs significantly from the VB case. Figures 4(b) to 4(d) show that the Gaussian beam is stretched at first, then a curved M-line appears corresponding to the resonant angle, and finally the Goos–Hänchen effect manifests itself as a discernible shift of the beam peak.
Figure 4.Evolution of the M-line in a reflected Gaussian beam under the same experimental conditions. The coupling angle of the resonant dip is around 9.13 deg. The intensity is normalized to the maximum of the reflected light spot in (b).
3. Theoretical Model
First, we demonstrate the high angular sensitivity of the UOMs, which is crucial to sensing the phase pattern of a VB. To clarify the inner relation among the UOMs, the surface plasmon (SP) mode, the symmetric (
Figure 5.Reflection spectra of a prism-coupled SMCW chip as a function of film thickness and incident angle. θc is the critical angle for total reflection of the guiding layer.
Let us further consider the finesse
Under weak coupling condition, there is
Next, we consider a Laguerre–Gaussian beam carrying an optical vortex with a topological charge
For the parameters,
Inserting Eq. (4) into Eq. (5) and neglecting the Gouy phase, we have
As shown in Fig. 6(a), it is simple to plot the distribution of
Figure 6.(a) Phase structure and related energy flux
When the beam is incident with a nonzero
For the calculation of the local incident angle
In view of Fig. 6(b), the normal to the chip surface
Eq. (8) can be applied to calculate the distribution of
4. Results and Discussion
As mentioned in Section 2, the outer rings in Fig. 2 are formed by random scattering of the natural roughness and the coupling between UOMs. The inner ring is formed mainly due to the direct reflection of the VB. Based on the proposed theory, the patterns of the inner ring can be simulated by taking the local incident angle
Figure 7.Simulated inner ring and the M-line of the reflected VB (l = 1) near a resonance, where the parameters are the same as those used in Fig.
The similarity between Figs. 2 and 7 is obvious, so the proposed model fits well with the experiments. Figure 7 shows the whole process of how the reflected beam is distorted by a twisted M-line. The M-line emerges from the central phase singularities, moves across the beam, and finally disappears into the singularity again. Since the M-line varies continuously as a function of the incident angle, the M-line method via UOMs provides sufficient sensitivity for the phase structure detection of the light beam carrying OAM.
For large incident angle
Figure 8.(a), (d) Intensities and (b), (e) phase structures of the reflected beams with different topological charge l; (c), (f) the phase structures of the incident beams are also plotted for comparison. The incident angle is θ = 30 deg, and the rest parameters are the same as those used in Fig.
5. Conclusion
In conclusion, we investigate the reflection of a VB by an SMCW chip. Owing to the high angular resolution of the UOMs, the phase structure of the reflected beam is well described by the evolution of twisted M-lines. A very simple model is proposed based on defining the local incident angle, and the theoretical prediction matches well with the experiments. This work provides an effective approach to the imaging and sensing of complicated phase structures.
References
[1] L. Allen, M. W. Beijersbergen, R. J. C. Spreeuw, J. P. Woerdman. Orbital angular momentum of light and the transformation of Laguerre–Gaussian laser modes. Phys. Rev. A, 45, 8185(1992).
[2] K. Y. Bliokh, F. J. Rodríguez-Fortuño, F. Nori, A. V. Zayats. Spin-orbit interactions of light. Nat. Photon., 9, 796(2015).
[3] M. Chen, M. Mazilu, Y. Arita, E. M. Wright, K. Dholakia. Dynamics of microparticles trapped in a perfect vortex beam. Opt. Lett., 38, 4919(2013).
[4] G. Xie, L. Li, Y. Ren, H. Huang, Y. Yan, N. Ahmed, Z. Zhao, M. P. J. Lavery, N. Ashrafi, S. Ashrafi, R. Bock, M. Tur, A. F. Molisch, A. E. Willner. Performance metrics and design considerations for a free-space optical orbital-angular-momentum–multiplexed communication link. Optica, 2, 357(2015).
[5] W. Qi, R. Liu, L. Kong, Z. Wang, S. Huang, C. Tu, Y. Li, H. Wang. Double-slit interference of single twisted photons. Chin. Opt. Lett., 18, 102601(2020).
[6] Y. Li, Y. Liu, X. Ling, X. Yi, X. Zhou, Y. Ke, H. Luo, S. Wen, D. Fan. Observation of photonic spin Hall effect with phase singularity at dielectric metasurfaces. Opt. Express, 23, 1767(2015).
[7] S. Zheng, J. Wang. On-chip orbital angular momentum modes generator and (de)multiplexer based on trench silicon waveguides. Opt. Express, 25, 18492(2017).
[8] P. F. Chimento, P. F. A. Alkemade, G. W. Hooft, E. R. Eliel. Optical angular momentum conversion in a nanoslit. Opt. Lett., 37, 4946(2012).
[9] Y. Yang, L. Wu, Y. Liu, D. Xie, Z. Jin, J. Li, G. Hu, C. Qiu. Deuterogenic plasmonic vortices. Nano. Lett., 20, 6774(2020).
[10] P. K. Tien, R. Ulrich, R. J. Martin. Modes of propagating light waves in thin deposited semiconductor films. Appl. Phys. Lett., 14, 291(1969).
[11] G. Chen, Z. Cao, J. Gu, Q. Shen. Oscillating wave sensors based on ultrahigh-order modes in symmetric metal-clad optical waveguides. Appl. Phys. Lett., 89, 081120(2006).
[12] J. Gu, G. Chen, Z. Cao, Q. Shen. An intensity measurement refractometer based on a symmetric metal-clad waveguide structure. J. Phys. D, 41, 2824(2008).
[13] H. Dai, L. Yuan, C. Yin, Z. Cao, X. Chen. Direct visualizing the spin Hall effect of light via ultrahigh-order modes. Phys. Rev. Lett., 124, 053902(2020).
[14] X. Zhou, L. Sheng, X. Ling. Photonic spin Hall effect enabled refractive index sensor using weak measurements. Sci. Rep., 8, 1221(2018).
[15] H. Dai, B. Jiang, C. Yin, Z. Cao, X. Chen. Ultralow-threshold continuous-wave lasing assisted by a metallic optofluidic cavity exploiting continuous pump. Opt. Lett., 43, 847(2018).
[16] X. Liu, Z. Cao, P. Zhu, Q. Shen, X. Liu. Large positive and negative lateral optical beam shift in prism-waveguide coupling system. Phys. Rev. E, 73, 056617(2006).
[17] L. Allen, M. Beijersbergen, R. Spreeuw, J. Woerdman. Orbital angular momentum of light and transformation of Laguerre Gaussian laser modes. Phys. Rev. A, 45, 8185(1992).
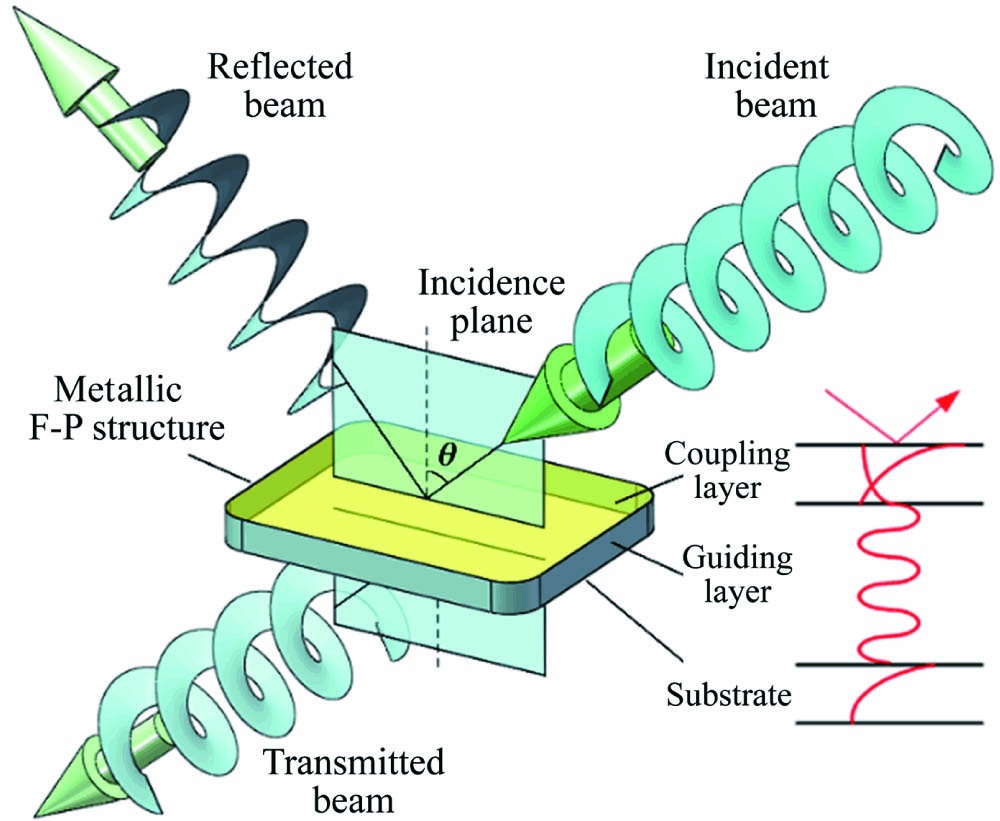
Set citation alerts for the article
Please enter your email address