
- Photonics Research
- Vol. 10, Issue 2, 456 (2022)
Abstract
1. INTRODUCTION
Over the last few decades, researchers have witnessed the emerging field of quantum information [1]. Various advances have been achieved in a plethora of hardware platforms [2,3]. A photon, due to its fast speed and robustness with respect to the thermal environment, is considered a perfect information carrier for quantum information processing [4,5]. Thus, nonclassical light sources, particularly indistinguishable correlated photon pairs, are a key resource for quantum communication [6,7] and quantum computation [8–11]. Besides these advances focusing on Fock-like states, squeezed light also serves as another fundamental resource for quantum information [12–15]. Parametric frequency conversion in nonlinear crystals is an indispensable approach in quantum optics to obtain nonclassical light [16,17], as well as to generate multi-photon entangled states [18–21].
To obtain efficient nonlinear interactions in bulk crystals, tight focusing is required. That, however, leads to small Rayleigh length, limiting the interaction volume. An alternative approach of using high pump powers may lead to undesired effects, including photorefraction and damage to the material. In the last few years, integrated photonics has stood out by offering a compact solution in the form of optical waveguides [22,23], which greatly reduces such undesirable effects by confining the pump light inside the waveguide, offering both tight focusing and large interaction volume at the same time. Recent progress in this field includes waveguide-based quantum sources implemented by femtosecond laser writing [24], UV-laser writing [25], and silicon photonics [26,27]. These platforms feature excellent generated photon brightness, purity, and low propagation loss on a chip. Recent work has shown the potential for an integrated source to encode information in both discrete and continuous variables [28].
To construct large-scale photonic quantum systems, high-quality building blocks should be integrated in a compact footprint with considerable complexity [29]. Compared to the silicon photonics platform, femtosecond laser writing chips show the capability of fully three-dimensional integration, which is suitable for efficient simulation of two-dimensional structures [30,31], and can be used to carry out various quantum information processing tasks [32–34]. When a nonclassical light source module is embedded into a complex on-chip arrangement, unwanted cross talk is prone to occur between adjacent waveguides due to the evanescent coupling. This cross talk not only modifies the generated quantum states of light, but also decreases the generation efficiency, since the pump light diverges into neighboring sites. The nonclassical features of the generated photons, for example, the photon-pair cross-correlations and the squeezing quality, can also decay because of this unwanted coupling. The key challenge is to protect efficient use of nonlinearity and quantumness of the generated photon pairs.
Sign up for Photonics Research TOC. Get the latest issue of Photonics Research delivered right to you!Sign up now
The topological phase allows protection of physical fields against unavoidable disorder. This effect was at the heart of recent demonstrations of topological protection for various nonlinear optical processes [35,36], including photon-pair generation [37–42]. The above, however, was studied only in the regime of low photon numbers, while strongly squeezed light remained out of scope despite the significant importance of squeezing in quantum optics. In this paper, we experimentally demonstrate the topological protection of squeezed light with a dimerized-type chain resembling Su–Schrieffer–Heeger (SSH) lattices on a photonic chip. We observe robust localization of topological states at different wavelengths. We verify the topological protection of quantum resources by measuring the transport dynamics such as light field distributions, cross-correlations, and squeezing by switching incident pump light between different input ports. Our results demonstrate that the topological protection is robust to the change in wavelength of nonclassical states, and can help to construct quantum squeezers in complex photonic circuits tolerant to fabrication imperfections.
2. EXPERIMENTS
Our topologically protected lattices are inscribed in a
Here,
The lattices for the topological protection of quantum light are composed of coupled waveguides with two sets of spacings, corresponding to the modulation of alternating couplings
Terms
Figure 1.Schematic diagram of the lattice with topological protection for squeezed light. (a) The structure of the lattice contains seven groups with lattice distances ranging from 5 mm to 35 mm. The short and long separations between adjacent waveguides are 7 μm and 9 μm, respectively. (b) Spectrum of the lattice. There are only two quasi zero-energy modes
We further illustrate the band diagram by characterizing the spectrum of the lattice, as shown in the Fig. 1(b). The band diagram contains two extended bands separated by the bandgap. Inside the bandgap, there are two modes
The experimental setup is schematically depicted in Fig. 2(a) (see Appendix F for experimental details). The mode-locked 780 nm femtosecond pump pulses (80 MHz) with vertical polarization (prepared by a combination of wave plates and a Glan–Taylor polarizer) can be injected into three different ports, namely, 1, 10, and 20. The output light intensity distributions of the pump light are accumulated by a CCD camera, and the evolution patterns under different distances varying from 5 mm to 35 mm [see I, II, and III in Fig. 2(b)] are also recorded. We experimentally verify the localization effect in the above three ports by comparing the measured pattern with theoretical simulation in Fig. 2(b). It is obvious that the pump light propagates locally as time evolves in the edge-state channel and the interface-defect channel (topological cases), while the beam diffuses and gradually couples to the adjacent sites in edge channel port 20 (trivial case). The localization effect in the topological channel ports indicates protection of the pump intensity, and provides sufficient power for generating photon pairs during FWM. The diffusion degrades the intensity as the propagation length increases, and the decreased pump intensity fails to achieve a high-quality quantum source during the FWM process. It can be deduced that the FWM in ports 1 and 10 is well protected at the wavelength of 780 nm. The pump light is spatially confined, and this localization makes FWM more efficient.
Figure 2.Experimental setup and intensity distributions of pump light. (a) Experimental setup for generation, filtering, and evolution measurement of nonclassical light. The input ports can be switched. DM, dichroic mirror; MCCM, multi-channel coincidence module. (b) Simulated intensity distributions of the pump light from ports 1, 10, and 20. The evolution distance is marked in white. (I), (II), and (III) show the experimentally measured intensity distributions from three input channel ports at 5 mm, 20 mm, and 35 mm evolution distances, respectively. The protected states from ports 1 and 10 always maintain localization as the propagation distance increases, while the unprotected state from port 20 diffuses over the lattice. The intensity distribution is normalized to its maximum.
After verifying the spatial protection of the pump light, we further test whether the on-chip generated correlated photons can also be protected. We first calculate the light intensity distributions of the signal and idler photons, and the theoretical simulation results are presented in Figs. 3(a) and 3(b), respectively. Even for light with different wavelengths, the localization effect still emerges in the topologically protected channels. To retrieve the signal and idler photons, we first filter out the residual pump light using both the polarization filter (Glan–Taylor polarizer) and spectrum filter (notch filters centered as 780 nm). Then, signal and idler photons are separated into two different spatial modes by a dichroic mirror, where idler photons are transmitted while signal photons are reflected. The photon pairs are coupled into single mode fibers, and then detected by avalanche photodiodes. All the coincidence counts are recorded by a homemade multi-channel coincidence module (MCCM). The experimental layout can be found in Fig. 2(a). To quantify the nonclassical features of the correlated photon pairs, we introduce the cross-correlation function as follows:
Figure 3.Performance of topological protection for the generated photon pairs. (a) Evolution of the signal photons generated with pump in different input ports. (b) Evolution of the idler photons generated with pump in different input ports. (c) Cross-correlations
In the FWM case, a value higher than two of the cross-correlation function
The cross-correlations
Due to the strong confinement of the pump light, our source can function as a squeezed light source [54] in the high-power regime. The threshold for optical damage, photorefraction, and other unwanted effects for fused silica is very high; thus, combined with the lattices for topological protection, both weak pump and strong pump regimes can be implemented. In the strong pump regime, the high-order terms of the photon-pair generation process are dominant, leading to the generation of squeezed light. In this work, we compare the squeezing parameters among three different structures. Squeezing parameter
As shown in Fig. 4, the squeezing parameters for the three channels are depicted in different colors under different evolution distances, where two distinct features can be observed. In the long evolution distance regimes, the squeezing parameters of the topologically protected channels are larger than that of the unprotected channels. In addition, the measured fluctuations using Possionian statistics in port 20 are greatly influenced by the beam diffusion unlike the topologically protected ports. These results indicate that the squeezed states can be well protected in the topological structure.
Figure 4.Experimental verification of topological protection for squeezed light. The squeezing parameters in ports 1, 10, and 20 are depicted in deep red, red, and blue, accordingly. The values of the squeezing parameters are more uniform and higher in two protected cases compared with unprotected port 20. The variance among three topological channels is different since unprotected port 20 fluctuates in a wider range. The inset shows the average fluctuation from each port. Compared to the results of the other two channel ports, channel port 20 is unprotected.
3. CONCLUSION
In conclusion, we have reported the topological protection of on-chip FWM and the generated squeezed light. By introducing a topological phase with a dimerized-type chain resembling SSH lattices on a silica photonic chip, we have observed localization and strong confinement of the pump light in topologically protected channels. The protected pump field fulfills the tight focusing condition to allow the waveguide to function as a high-quality quantum squeezer. We have demonstrated that the protection applies to different wavelengths, impacting the cross-correlations and squeezing parameters. It showcases a robust generation of quantum resources for future practical quantum information tasks, such as Gaussian boson sampling and bosonic error correction. We have verified the validity of the topological protection of on-chip squeezers, which may play an essential role in photonic quantum information processing (see Appendix C for discussions of robustness and validity) [55].
Acknowledgment
Acknowledgment. X.-M.J. acknowledges additional support from a Shanghai talent program and support from Zhiyuan Innovative Research Center of Shanghai Jiao Tong University.
APPENDIX A: CALCULATION OF THE SQUEEZING PARAMETER
In this paper, we adopt the Oxford group’s method to evaluate the squeezing parameter [
We measured the squeezing parameter
As shown in Fig.
Figure 5.Experimental schematic of measuring the heralded second-order correlation
The heralding efficiency
As can be inferred from Eqs. (
We can obtain other terms in a similar way. Then we multiply these terms together and finally obtain the squeezing parameter:
Regarding the fluctuation in Fig.
The fluctuation of the detected photon number is governed by Poisson statistics.
APPENDIX B: HAMILTONIAN OF TOPOLOGICALLY PROTECTED QUANTUM LIGHT LATTICES
As shown in Fig.
Figure 6.Sketch of lattices for topological protection of squeezed light. There are 20 sites and 10 unit cells in the lattice. The red line marks the large spacing 9 μm with weak coupling of
APPENDIX C: ROBUSTNESS AND VALIDITY OF TOPOLOGICAL MODULES
In this section, we explain the motivation for topologically protected quantum light sources. Our work realizes the protection of squeezed light by a topological lattice. It is applicable to large-scale photonic structures where unwanted cross talk can pose a significant problem. Our method for protecting squeezed light may be practical in the following future scenarios. System.Xml.XmlElementSystem.Xml.XmlElement
To further verify the robustness and validity of our model, we design and simulate different topological modules to test the cross talk effect in a one-dimensional lattice. As shown in Figs.
Figure 7.Verification of topological protection effect provided by topological modules. We design two topological structures containing ports 1 and 10 from the main text, and a module containing port 20 is considered as a comparison. These modules are embedded into equally spaced arrays (
The uniform-spacing lattices are chosen to have three different spacings, as Figs.
APPENDIX D: MULTI-MODE ANALYSIS OF FWM PROCESS
We can calculate the source joint spectral amplitude (JSA) using the following expression:
Here,
Using Schmidt decomposition on the JSA, we can obtain
Experimentally, we probe
In the low-gain regime (pump power is 5 mW), the measured results are
APPENDIX E: ESTIMATION OF THE MODE FIELD DIAMETER
To estimate the mode field diameter of the fabricated waveguides, we extract the intensity distribution of each single light spot from the data collected by the CCD. One of the light spots is shown in Fig.
Figure 8.Estimation of the mode field diameter. The intensity distributions of the light spots are extracted from the CCD data and fitted by 2D Gaussian functions. The beam diameters in
Through fitting, we can calculate the pixel numbers of the horizontal and vertical field diameters at
To reduce errors in the estimation process, we collect the intensity distribution of 18 light spots from three different groups, and calculate the average mode field diameter, which is
APPENDIX F: EXPERIMENTAL DETAILS OF THE PHOTONIC CHIP AND THE MEASUREMENT SETUP
In our experiment, we use an ultrafast Ti:sapphire oscillator centered at 780 nm as the pump light. The generated signal and idler photon pairs are first separated by a dichroic mirror and three cascaded notch filters (Semrock, NF03-785E-25). Then, in both signal and idler photon arms, additional filters (Semrock, FF01-770/SP-25 and Semrock, BLP01-785R-25) are added to suppress the noise photons.
We also measure the spectra of both signal and idler photons, and the central wavelengths are 732.5 nm and 834.3 nm, respectively. The measured spectra are shown in Fig.
Figure 9.Measured spectra of signal and idler photons.
The collection efficiencies for the signal and idler photons into the optical fibers are almost the same, all beyond 85%. Through the subsequent on-chip filtering and further plans for “all on-chip project,” we believe the coupling loss can be further decreased. However, in our current work, the relatively small photon losses do not have a strong impact on our results.
References
[1] M. Nielsen, I. Chuang. Quantum Computation and Quantum Information(2000).
[2] T. D. Ladd, F. Jelezko, R. Laflamme, Y. Nakamura, C. Monroe, J. L. O’Brien. Quantum computers. Nature, 464, 45-53(2010).
[3] G. Popkin. Quest for qubits. Science, 354, 1090-1093(2016).
[4] L. Mandel, E. Wolf. Optical Coherence and Quantum Optics(1995).
[5] J. L. O’Brien, A. Furusawa, J. Vučković. Photonic quantum technologies. Nat. Photonics, 3, 687-695(2009).
[6] N. Gisin, G. Ribordy, W. Tittel, H. Zbinden. Quantum cryptography. Rev. Mod. Phys., 74, 145-195(2002).
[7] F. Xu, X. Ma, Q. Zhang, H.-K. Lo, J.-W. Pan. Secure quantum key distribution with realistic devices. Rev. Mod. Phys., 92, 025002(2020).
[8] C. K. Hong, Z. Y. Ou, L. Mandel. Measurement of subpicosecond time intervals between two photons by interference. Phys. Rev. Lett., 59, 2044-2046(1987).
[9] P. Kok, W. J. Munro, K. Nemoto, T. C. Ralph, J. P. Dowling, G. J. Milburn. Linear optical quantum computing with photonic qubits. Rev. Mod. Phys., 79, 135-174(2007).
[10] M. Lebugle, M. Gräfe, R. Heilmann, A. Perez-Leija, S. Nolte, A. Szameit. Experimental observation of N00N state Bloch oscillations. Nat. Commun., 6, 8273(2015).
[11] C. Sparrow, E. Martín-López, N. Maraviglia, A. Neville, C. Harrold, J. Carolan, Y. N. Joglekar, T. Hashimoto, N. Matsuda, J. L. O’Brien, D. P. Tew, A. Laing. Simulating the vibrational quantum dynamics of molecules using photonics. Nature, 557, 660-667(2018).
[12] S. L. Braunstein, P. van Loock. Quantum information with continuous variables. Rev. Mod. Phys., 77, 513-577(2005).
[13] C. Weedbrook, S. Pirandola, R. García-Patrón, N. J. Cerf, T. C. Ralph, J. H. Shapiro, S. Lloyd. Gaussian quantum information. Rev. Mod. Phys., 84, 621-669(2012).
[14] C. S. Hamilton, R. Kruse, L. Sansoni, S. Barkhofen, C. Silberhorn, I. Jex. Gaussian Boson sampling. Phys. Rev. Lett., 119, 170501(2017).
[15] D. Gottesman, A. Kitaev, J. Preskill. Encoding a qubit in an oscillator. Phys. Rev. A, 64, 012310(2001).
[16] C. Kurtsiefer, M. Oberparleiter, H. Weinfurter. Generation of correlated photon pairs in type-II parametric down conversion-revisited. J. Mod. Opt., 48, 1997-2007(2001).
[17] L. Caspani, C. Xiong, B. J. Eggleton, D. Bajoni, M. Liscidini, M. Galli, R. Morandotti, D. J. Moss. Integrated sources of photon quantum states based on nonlinear optics. Light Sci. Appl., 6, e17100(2017).
[18] P. G. Kwiat, K. Mattle, H. Weinfurter, A. Zeilinger, A. V. Sergienko, Y. Shih. New high-intensity source of polarization-entangled photon pairs. Phys. Rev. Lett., 75, 4337-4341(1995).
[19] J.-W. Pan, M. Daniell, S. Gasparoni, G. Weihs, A. Zeilinger. Experimental demonstration of four-photon entanglement and high-fidelity teleportation. Phys. Rev. Lett., 86, 4435-4438(2001).
[20] X.-L. Wang, L.-K. Chen, W. Li, H.-L. Huang, C. Liu, C. Chen, Y.-H. Luo, Z.-E. Su, D. Wu, Z.-D. Li, H. Lu, Y. Hu, X. Jiang, C.-Z. Peng, L. Li, N.-L. Liu, Y.-A. Chen, C.-Y. Lu, J.-W. Pan. Experimental ten-photon entanglement. Phys. Rev. Lett., 117, 210502(2016).
[21] H.-S. Zhong, Y. Li, W. Li, L.-C. Peng, Z.-E. Su, Y. Hu, Y.-M. He, X. Ding, W. Zhang, H. Li, L. Zhang, Z. Wang, L. You, X.-L. Wang, X. Jiang, L. Li, Y.-A. Chen, N.-L. Liu, C.-Y. Lu, J.-W. Pan. 12-photon entanglement and scalable scattershot boson sampling with optimal entangled-photon pairs from parametric down-conversion. Phys. Rev. Lett., 121, 250505(2018).
[22] J.-W. Wang, F. Sciarrino, A. Laing, M. G. Thompson. Integrated photonic quantum technologies. Nat. Photonics, 14, 273-284(2020).
[23] F. Flamini, N. Spagnolo, F. Sciarrino. Photonic quantum information processing: a review. Rep. Prog. Phys., 82, 016001(2019).
[24] J. B. Spring, P. S. Salter, B. J. Metcalf, P. C. Humphreys, M. Moore, N. Thomas-Peter, M. Barbieri, X.-M. Jin, N. K. Langford, W. S. Kolthammer, M. J. Booth, I. A. Walmsley. On-chip low loss heralded source of pure single photons. Opt. Express, 21, 13522-13532(2013).
[25] J. B. Spring, P. L. Mennea, B. J. Metcalf, P. C. Humphreys, J. C. Gates, H. L. Rogers, C. Söller, B. J. Smith, W. S. Kolthammer, P. G. R. Smith, I. A. Walmsley. A chip-based array of near-identical, pure, heralded single photon sources. Optica, 4, 90-96(2017).
[26] J. W. Silverstone, D. Bonneau, K. Ohira, N. Suzuki, H. Yoshida, N. Iizuka, M. Ezaki, C. M. Natarajan, M. G. Tanner, R. H. Hadfield, V. Zwiller, G. D. Marshall, J. G. Rarity, J. L. O’Brien, M. G. Thompson. On-chip quantum interference between silicon photon-pair sources. Nat. Photonics, 8, 104-108(2014).
[27] S. Paesani, M. Borghi, S. Signorini, A. Maïnos, L. Pavesi, A. Laing. Near-ideal spontaneous photon sources in silicon quantum photonics. Nat. Commun., 11, 2505(2020).
[28] R.-J. Ren, J. Gao, W.-H. Zhou, Z.-Q. Jiao, L.-F. Qiao, X.-W. Wang, X.-M. Jin. 128 identical quantum sources integrated on a single silica chip. Phys. Rev. Applied, 16, 054026(2021).
[29] J.-W. Wang, S. Paesani, Y. Ding, R. Santagati, P. Skrzypczyk, A. Salavrakos, J. Tura, R. Augusiak, L. Mančinska, D. Bacco, D. Bonneau, J. W. Silverstone, Q. Gong, A. Acín, K. Rottwitt, L. K. Oxenløwe, J. L. O’Brien, A. Laing, M. G. Thompson. Multidimensional quantum entanglement with large-scale integrated optics. Science, 360, 285-291(2018).
[30] K. M. Davis, K. Miura, N. Sugimoto, K. Hirao. Writing waveguides in glass with a femtosecond laser. Opt. Lett., 21, 1729-1731(1996).
[31] R. Osellame, G. Cerullo, R. Ramponi. Femtosecond Laser Micromachining: Photonic and Microfluidic Devices in Transparent Materials(2012).
[32] H. Tang, X.-F. Lin, Z. Feng, J.-Y. Chen, J. Gao, K. Sun, C.-Y. Wang, P.-C. Lai, X.-Y. Xu, Y. Wang, L.-F. Qiao, A.-L. Yang, X.-M. Jin. Experimental two-dimensional quantum walk on a photonic chip. Sci. Adv., 4, eaat3174(2018).
[33] H. Tang, C. D. Franco, Z.-Y. Shi, T.-S. He, Z. Feng, J. Gao, K. Sun, Z.-M. Li, Z.-Q. Jiao, T.-Y. Wang, M. S. Kim, X.-M. Jin. Experimental quantum fast hitting on hexagonal graphs. Nat. Photonics, 12, 754-758(2018).
[34] Z.-Q. Jiao, J. Gao, W.-H. Zhou, X.-W. Wang, R.-J. Ren, X.-Y. Xu, L.-F. Qiao, Y. Wang, X.-M. Jin. Two-dimensional quantum walk of correlated photons. Optica, 8, 1129-1135(2021).
[35] D. Leykam, A. S. Solntsev, A. A. Sukhorukov, A. S. Desyatnikov. Lattice topology and spontaneous parametric down-conversion in quadratic nonlinear waveguide arrays. Phys. Rev. A, 92, 033815(2015).
[36] D. Smirnova, D. Leykam, Y. Chong, Y. Kivshar. Nonlinear topological photonics. Appl. Phys. Rev., 7, 021306(2020).
[37] A. Blanco-Redondo, B. Bell, D. Oren, B. J. Eggleton, M. Segev. Topological protection of biphoton states. Science, 362, 568-571(2018).
[38] M. Wang, C. Doyle, B. Bell, M. J. Collins, E. Magi, B. J. Eggleton, M. Segev, A. Blanco-Redondo. Topologically protected entangled photonic states. Nanophotonics, 8, 1327-1335(2019).
[39] K. Tschernig, Á. Jimenez-Galán, D. N. Christodoulides, M. Ivanov, K. Busch, M. A. Bandres, A. Perez-Leija. Topological protection versus degree of entanglement of two-photon light in photonic topological insulators. Nat. Commun., 12, 1974(2021).
[40] M. C. Rechtsman, Y. Lumer, Y. Plotnik, A. Perez-Leija, A. Szameit, M. Segev. Topological protection of photonic path entanglement. Optica, 3, 925-930(2016).
[41] Y. Wang, X.-L. Pang, Y.-H. Lu, J. Gao, Y.-J. Chang, L.-F. Qiao, Z.-Q. Jiao, H. Tang, X.-M. Jin. Topological protection of two-photon quantum correlation on a photonic chip. Optica, 6, 955-960(2019).
[42] Y. Wang, Y.-H. Lu, J. Gao, R.-J. Ren, Y.-J. Chang, Z.-Q. Jiao, Z.-Y. Zhang, X.-M. Jin. Topologically protected quantum entanglement(2019).
[43] W. P. Su, J. R. Schrieffer, A. J. Heeger. Solitons in polyacetylene. Phys. Rev. Lett., 42, 1698-1701(1979).
[44] L. Lu, J. D. Joannopoulos, M. Soljacic. Topological photonics. Nat. Photonics, 8, 821-829(2014).
[45] T. Ozawa, H. M. Price, A. Amo, N. Goldman, M. Hafezi, L. Lu, M. C. Rechtsman, D. Schuster, J. Simon, O. Zilberberg, I. Carusotto. Topological photonics. Rev. Mod. Phys., 91, 015006(2019).
[46] A. Blanco-Redondo, I. Andonegui, M. J. Collins, G. Harari, Y. Lumer, M. C. Rechtsman, B. J. Eggleton, M. Segev. Topological optical waveguiding in silicon and the transition between topological and trivial defect states. Phys. Rev. Lett., 116, 163901(2016).
[47] J. Zak. Berry’s phase for energy bands in solids. Phys. Rev. Lett., 62, 2747-2750(1989).
[48] P. Delplace, D. Ullmo, G. Montambaux. Zak phase and the existence of edge states in graphene. Phys. Rev. B, 84, 195452(2011).
[49] J. Noh, W. A. Benalcazar, S. Huang, M. J. Collins, K. P. Chen, T. L. Hughes, M. C. Rechtsman. Topological protection of photonic mid-gap defect modes. Nat. Photonics, 12, 408-415(2018).
[50] A. Moroz. Minima and maxima of the local density of states for one-dimensional periodic systems. Europhys. Lett., 46, 419-424(1999).
[51] M. Verbin, O. Zilberberg, Y. E. Kraus, Y. Lahini, Y. Silberberg. Observation of topological phase transitions in photonic quasicrystals. Phys. Rev. Lett., 110, 076403(2013).
[52] H. de Riedmatten, J. Laurat, C. W. Chou, E. W. Schomburg, D. Felinto, H. J. Kimble. Direct measurement of decoherence for entanglement between a photon and stored atomic excitation. Phys. Rev. Lett., 97, 113603(2006).
[53] A. Christ, K. Laiho, A. Eckstein, K. N. Cassemiro, C. Silberhorn. Probing multimode squeezing with correlation functions. New J. Phys., 13, 033027(2011).
[54] D. L. Andrews, A. I. Lvovsky. Squeezed light. Photonics(2015).
[55] J. M. Arrazola, V. Bergholm, K. Brádler, T. R. Bromley, M. J. Collins, I. Dhand, A. Fumagalli, T. Gerrits, A. Goussev, L. G. Helt, J. Hundal, T. Isacsson, R. B. Israel, J. Izaac, S. Jahangiri, R. Janik, N. Killoran, S. P. Kumar, J. Lavoie, A. E. Lita, D. H. Mahler, M. Menotti, B. Morrison, S. W. Nam, L. Neuhaus, H. Y. Qi, N. Quesada, A. Repingon, K. K. Sabapathy, M. Schuld, D. Su, J. Swinarton, A. Száva, K. Tan, P. Tan, V. D. Vaidya, Z. Vernon, Z. Zabaneh, Y. Zhang. Quantum circuits with many photons on a programmable nanophotonic chip. Nature, 591, 54-60(2021).
[56] A. Szameit, F. Dreisow, T. Pertsch, S. Nolte, A. Tünnermann. Control of directional evanescent coupling in fs laser written waveguides. Opt. Express, 15, 1579-1587(2007).
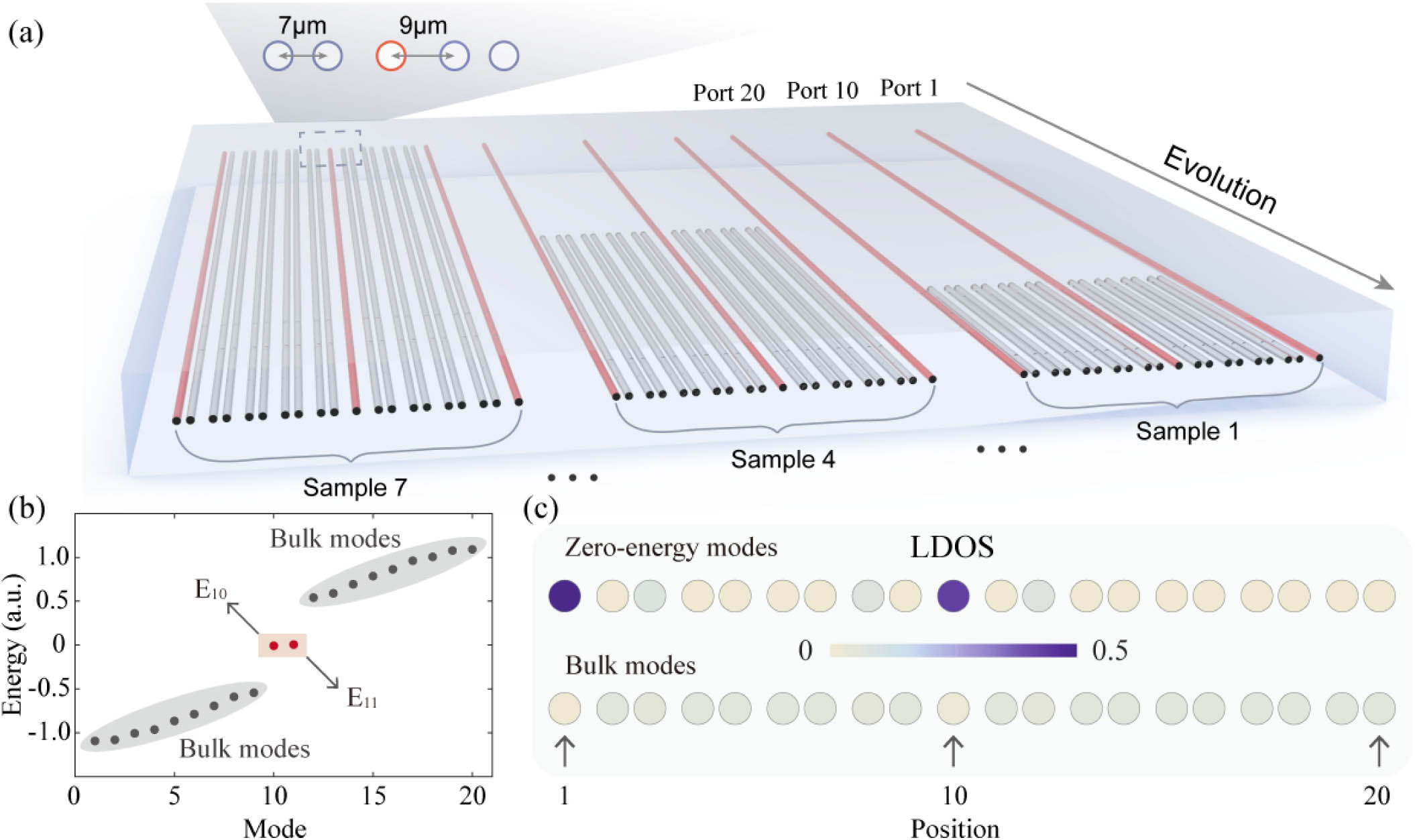
Set citation alerts for the article
Please enter your email address