
- Chinese Optics Letters
- Vol. 19, Issue 7, 071701 (2021)
Abstract
1. Introduction
Photoacoustic microscopy (PAM) is a hybrid biomedical imaging technology that has the capability of providing efficient optical contrast, utilizing a configuration of optical illumination and ultrasonic detection[
With the advancement of semiconductor technology, the laser diode (LD) as an excitation source is conducive to achieving the miniaturization and integration of system light sources, which offers broad prospects because of its advantages such as small size, low cost, commercial availability, and stable performance[
A reflective objective lens is currently the most promising method to get a long WD[
Sign up for Chinese Optics Letters TOC. Get the latest issue of Chinese Optics Letters delivered right to you!Sign up now
2. Materials and Methods
To obtain a highly detectable photoacoustic signal, modulating the commercial LD [Fig. 1(a)] through driving circuits to get the pulse mode is necessary. In this paper, the pulse width modulation of the drive circuit is obtained by a built-in program single-chip microcomputer (STM32F405, STMicroelectronics) and an operational amplifier (LM358, National Semiconductor). The driving circuit of the LD is based on the principle of a constant current source circuit, and the voltage stabilizing circuit is composed mainly of a synchronous step-down voltage regulator (MP2303ADN, MPS) driven by STM32F405, as shown in Fig. 1(b). To get 10 V output, element parameters used in the drive circuit are
Figure 1.(a) Photograph of the LD, (b) schematic diagram of the stabilization drive circuit of pulse LD, (c) measured pulse width of the LD where the trigger signal and pulse driving signal are delayed by 120 ns, and (d) exported energy stability test generated by each pulse of the excitation source.
Figure 2(a) shows the experimental configuration and schematic of the compact LD-OR-PAM system. The photoacoustic excitation source is a 450 nm LD with a pulse repetition frequency of 1 kHz. The pulsed beam from the LD, whose spot shape is a rectangle of
Figure 2.(a) The left is the photograph of system, and the right is the schematic diagram of the LD-OR-PAM system, (b) relationship of spot size and the distance between the reflective objective and spherical lens in the LD-OR-PAM system, and (c) the photoacoustic amplitude and energy density distribution of the sample absorption source along the z axis.
The LD-OR-PAM system uses a water-immersed ultrasonic transducer (N0.5P28L, DOPPLER) with a center frequency of 0.5 MHz and a
3. Results
To measure the lateral resolution of the LD-OR-PAM system experimentally, a sharp edge in a United States Air Force (USAF) resolution test target (GCG-02062, Daheng Optics) with pairs of longitudinal and lateral rectangular stripes with different sizes was divided into six groups with six element sizes. In this experiment, the imaged bars belonged to group 4 and element 1. Figure 3(a) presents photoacoustic reconstructed images of a USAF target, which is highlighted with a 2 µm step size. The geometric shape and relative position of the stripes on the USAF target could be reconstructed clearly by the LD-OR-PAM system. As shown in Fig. 3(b), the image at
Figure 3.(a) Reconstructed image of a USAF (4-1) and (b) assessed lateral resolution of the LD-OR-PAM system along the dotted line of (a).
To demonstrate the performance of the LD-OR-PAM system, a few irregularly arranged carbon fibers (diameter: ∼7 µm) used to simulate small capillary-sized blood vessels were imaged with a smaller field of view. The carbon fibers were suspended in the ultrasound gel and averaged 64 times with an optimized SNR in the experiment. Figure 4(a) shows the reconstructed image of carbon fibers with a step size of 3 µm. In order to calculate the SNR of the photoacoustic image, the area in the image that only contains background noise was selected to obtain averaged noise amplitude. It was observed that the position and profile of five carbon fibers can be clearly distinguished, and the effective photoacoustic signal has an upper SNR of 21 dB. Next, several black hairs were regularly arranged in a certain area with a step size of 50 µm. As is shown in Fig. 4(b), the structure of the hairs can be clearly presented, where the hairs’ breaking points are partly because of bubbles in the coupling fluid. The peak-to-peak SNR of the photoacoustic signal is about 17 dB. These results demonstrate that the LD-OR-PAM system has the capability of imaging tissue with biological light absorption.
Figure 4.(a) Photoacoustic image of five carbon fibers and (b) photoacoustic image of crossed hair strands.
To further validate the label-free imaging feasibility of the system in vivo, we imaged subcutaneous microvascular networks in a mouse ear. Before the experiment, hairs of the mouse [Fig. 5(a)] were chemically depilated, and a thin layer of ultrasound gel was applied to the mouse skin. All animal experimental procedures were performed in accordance with the guidelines of the Guangdong University of Technology. The imaging area was
Figure 5.(a) Photograph of subcutaneous microvascular networks in a mouse ear, (b) the acquired 2D photoacoustic image of the subcutaneous microvascular structure in vivo, and (c) the vessel diameter at the dotted line in (b) is estimated.
Figure 6.(a) Photoacoustic image of another subcutaneous microvascular structure and (b) photoacoustic image of the same area as in (a) but at different depths.
4. Conclusion
Most VIS LDs currently provide a peak output power of only hundreds of milliwatts, which is about three orders of magnitude less than that of NIR LDs. Compared with other VIS LDs, the highest power available for a 450 nm commercial pulsed LD is about 2 W according to research. Using a 450 nm LD may potentially enhance the lateral resolution of the PAM system, and the absorption coefficient of hemoglobin in the VIS light band is several orders of magnitude higher than that in the NIR light band. Meanwhile, pulsed lasers are much more frequently used in PAM, mainly because the SNR of the photoacoustic signal in pulsed-laser excitation is greater than that in a continuous-wave laser. According to the maximum allowable contact limit of the American National Standards Institute[
In the experiment, the acquired data is averaged 64 times using a pulsed LD with 1 kHz repetition frequency and a PXIe-5105 data acquisition card, which can acquire 6–7 points per second. Scanning an area of
As a possibility, a focused ring-shaped ultrasonic transducer used in a customized way that has a central hole is coaxially aligned with the plane-convex lens. Since the laser beam needs to pass through the hollow transducer, a larger spatial position is usually required to ensure that the beam is not blocked, which may cause a certain direction angle between excitation position of the photoacoustic signal and the receiving position of the transducer. The detection sensitivity of the hollow transducer decreases due to the large angle at which the photoacoustic signal is received. What is more, there is at least a 10% loss of acoustic energy due to a mismatch in the receiving angle and reduced acoustic focus due to the presence of the central hole[
In summary, a compact VIS LD-OR-PAM system with a reflective objective to achieve a 22 mm long WD and a 10 µm lateral resolution is used. Different depth subcutaneous microvascular networks in label-free mouse ears have successfully reappeared in vivo with an acceptable SNR by confocal alignment. It will be a promising tool to develop into a handy tool for subcutaneous blood vessel imaging. Currently, the LD-OR-PAM system adopts the transmission mode, because the miniaturization of the low-frequency ultrasonic transducer is still an international problem in engineering, which is a trade-off between size and center frequency. We are trying to use a stereolithography technology[
References
[1] J. Yao, L. Wang, J. M. Yang, K. I. Maslov, T. T. W. Wong, L. Li, C.-H. Huang, J. Zou, L. H. V. Wang. High-speed label-free functional photoacoustic microscopy of mouse brain in action. Nat. Methods, 12, 407(2015).
[2] P. F. Hai, T. Imai, S. Xu, R. Y. Zhang, R. L. Aft, J. Zou, L. H. V. Wang. High-throughput, label-free, single-cell photoacoustic microscopy of intratumoral metabolic heterogeneity. Nat. Biomed. Eng., 3, 381(2019).
[3] J. Kim, J. Y. Kim, S. Jeon, J. W. Baik, S.H. Cho, C. Kim. Super-resolution localization photoacoustic microscopy using intrinsic red blood cells as contrast absorbers. Light: Sci. Appl., 8, 103(2019).
[4] W. Y. Zhang, H. G. Ma, Z. W. Cheng, Z. Y. Wang, K. D. Xiong, S. H. Yang. High-speed dual-view photoacoustic imaging pen. Opt. Lett., 45, 1599(2020).
[5] J. B. Tang, X. J. Dai, H. B. Jiang. Wearable scanning photoacoustic brain imaging in behaving rats. J. Biophoton., 9, 570(2016).
[6] T. Jin, H. Guo, H. B. Jiang, B. W. Ke, L. Xi. Portable optical resolution photoacoustic microscopy (pORPAM) for human oral imaging. Opt. Lett., 42, 4434(2017).
[7] Q. Chen, H. Guo, T. Jin, W. Z. Qi, H. K. Xie, L. Xi. Ultracompact high-resolution photoacoustic microscopy. Opt. Lett., 43, 1615(2018).
[8] P. Hajireza, W. Shi, R. J. Zemp. Real-time handheld optical-resolution photoacoustic microscopy. Opt. Express, 19, 20097(2011).
[9] F. Duan, H. Ma, J. Zhang, S. Li, H. Li, Z. Wu, F. Hong, L. Zeng, L. Nie. Optical-resolution photoacoustic microscopy continually monitors macrophages activities of acute inflammation in vivo. Chin. Opt. Lett., 18, 121701(2020).
[10] X. Wang, K. Xiong, X. Jin, S. Yang. Tomography-assisted Doppler photoacoustic microscopy: proof of concept. Chin. Opt. Lett., 18, 101702(2020).
[11] L. Zeng, G. Liu, D. Yang, X. Ji. 3D-visual laser-diode-based photoacoustic imaging. Opt. Express, 20, 1237(2012).
[12] H. T. Zhong, J. Y. Zhang, T. Y. Duan, H. R. Lan, M. Zhou, F. Gao. Enabling both time-domain and frequency-domain photoacoustic imaging by a fingertip laser diode system. Opt. Lett., 44, 1988(2019).
[13] T. H. Wang, S. Nandy, H. S. Salehi, P. D. Kumavor, Q. Zhu. A low-cost photoacoustic microscopy system with a laser diode excitation. Biomed. Opt. Express, 5, 3053(2014).
[14] P. K. Upputuri, M. Pramanik. Performance characterization of low-cost, high-speed, portable pulsed laser diode photoacoustic tomography (PLD-PAT) system. Biomed. Opt. Express, 6, 4118(2015).
[15] A. Stylogiannis, L. Prade, A. Buehler, J. Aguirre, V. Ntziachristos. Continuous wave laser diodes enable fast optoacoustic imaging. Photoacoustics, 9, 31(2018).
[16] W. Xia, M. K. A. Singh, E. Maneas, N. Sato, Y. Shigeta, T. Agano, S. Ourselin, S. J. West, A. E. Desjardins. Handheld real-time LED-based photoacoustic and ultrasound imaging system for accurate visualization of clinical metal needles and superficial vasculature to guide minimally invasive procedures. Sensors, 18, 1394(2018).
[17] L. Zeng, G. Liu, D. Yang, X. Ji. Cost-efficient laser-diode-induced optical-resolution photoacoustic microscopy for two-dimensional/three-dimensional biomedical imaging. J. Biomed. Opt., 19, 076017(2014).
[18] L. Zeng, Z. Piao, S. Huang, W. Jia, Z. Chen. Label-free optical-resolution photoacoustic microscopy of superficial microvasculature using a compact visible laser diode excitation. Opt. Express, 23, 31026(2015).
[19] J. Yao, L. V. Wang. Sensitivity of photoacoustic microscopy. Photoacoustics, 2, 87(2014).
[20] L. V. Wang, S. Hu. Photoacoustic tomography: in vivo imaging from organelles to organs. Science, 335, 1458(2012).
[21] X. J. Dai, H. Yang, H. B. Jiang. In vivo photoacoustic imaging of vasculature with a low-cost miniature light emitting diode excitation. Opt. Lett., 42, 1456(2017).
[22] M. Erfanzadeh, Q. Zhu. Photoacoustic imaging with low-cost sources: a review. Photoacoustics, 14, 1(2019).
[23] S. K. Kalva, P. K. Upputuri, M. Pramanik. High-speed, low-cost, pulsed-laser-diode-based second-generation desktop photoacoustic tomography system. Opt. Lett., 44, 81(2019).
[24] L. Zeng, G. Liu, D. Yang, X. Ji. Portable optical-resolution photoacoustic microscopy with a pulsed laser diode excitation. Appl. Phys. Lett., 102, 053704(2013).
[25] Q. Yao, Y. Ding, G. Liu, L. Zeng. Low-cost photoacoustic imaging systems based on laser diode and light-emitting diode excitation. J. Innov. Opt. Health Sci., 10, 1730003(2017).
[26] R. Cao, J. P. Kilroy, B. Ning, T. X. Wang, J. A. Hossack, S. Hu. Multispectral photoacoustic microscopy based on an optical–acoustic objective. Photoacoustics, 3, 55(2015).
[27] H. Wang, X. Yang, Y. Liu, B. Jiang, Q. Luo. Reflection-mode optical-resolution photoacoustic microscopy based on a reflective objective. Opt. Express, 21, 24210(2013).
[28] C. Zhang, K. Maslov, J. Yao, L. V. Wang. In vivo photoacoustic microscopy with 7.6-µm axial resolution using a commercial 125-MHz ultrasonic transducer. J. Biomed. Opt., 17, 116016(2012).
[29] . ANSI Z136.1–2007: American National Standard for Safe Use of Lasers(2007).
[30] M. Erfanzadeh, P. D. Kumavor, Q. Zhu. Laser scanning laser diode photoacoustic microscopy system. Photoacoustics, 9, 1(2018).
[31] J. Cheng, Y. Chen, J.-W. Wu, X.-R. Ji, S.-H. Wu. 3D printing of BaTiO3 piezoelectric ceramics for a focused ultrasonic array. Sensors, 19(2019).
[32] Y. Chen, X. Bao, C.-M. Wong, J. Cheng, H. Wu, H. Song, X. Ji, S. Wu. PZT ceramics fabricated based on stereolithography for an ultrasound transducer array application. Ceram. Int., 44, 22725(2018).
[33] T. J. Allen, P. C. Beard. Dual wavelength laser diode excitation source for 2D photoacoustic imaging. Proc. SPIE, 6437, 64371U(2007).
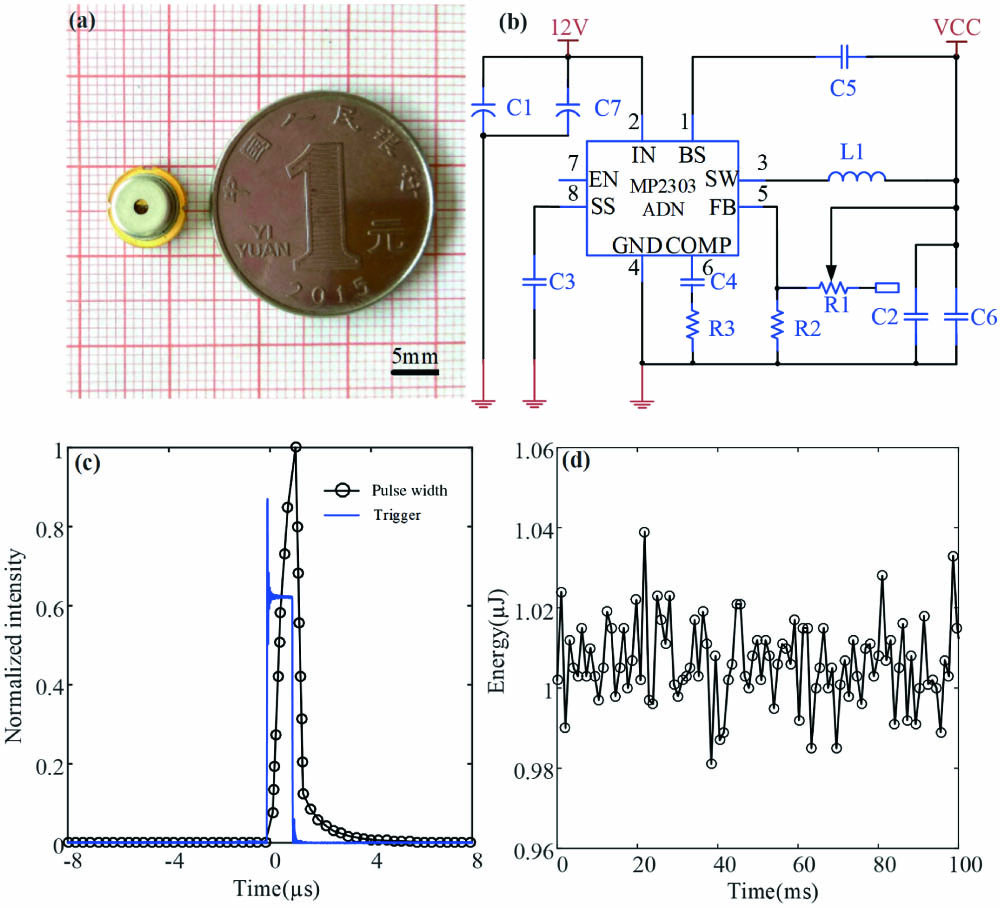
Set citation alerts for the article
Please enter your email address