Abstract
This paper reports a coherent random microcavity laser that consists of a disordered cladding (scattering) layer and a light-amplification core filled with dye solution. Cold cavity analysis indicates that the random resonance modes supported by the proposed cavity can be effectively excited. With introducing the gain material, random lasing by specific modes is observed to show typical features of coherent random lasers, such as spatially incoherent emission of random modes. By inserting a metal nanoparticle into the gain region, emission wavelength/intensity of the random lasers can be considerably tuned by changing the position of the inserted nanoparticle, opening up new avenues for controlling output of random lasers and sensing applications (e.g., small particle identification, location, etc.).1. INTRODUCTION
Light scattering is a daily phenomenon that is sometimes detrimental to scientific application, e.g., it leads to higher photon loss in conventional laser systems. However, light scattering could be beneficial for lasing systems where light is generated in a nanostructured scattering environment or in systems where a high-quality cavity is difficult to be made through point mirrors [1–3]. Over the past decades, random lasers, generated through the amplified stimulated emission of light scattering, have attracted much attention in systems, including photonic crystals, polymeric matrices, dye-solution-doped with nano-powders, semiconductors, quantum dots, cold atoms, biological tissues, optical fibers [4–15], etc.
Several kinds of random laser systems have been reported so far: for example, random lasers formed by one kind of material that can function as scatter and gain material and others formed by a kind of gain material and another kind of scattering media [13–16]. For the second case, when the gain media is mixed with scattering media, the scattering process can be easily modulated by changing the types and concentration of the scattering nanoparticle. When the gain and scattering media are independent, light amplification and the scattering process can be separately controlled, which facilitates control and stabilization of output. Up to now, this type of random laser has been reported in systems such as photonic crystal membranes with engineered disorders, polymer waveguides embodied by scattering boundaries, and a fiber-type waveguide with a disordered cladding layer [17–19].
Here, we propose a design of a random microcavity laser with a decoupled gain and scattering region, which is formed by a cladding layer for light scattering and a core area for light amplification filled with a dye solution. Through cold cavity analysis, localized modes of the proposed cavity are found, and the random lasing character of the laser is analyzed by introducing a gain mechanism in the core area. Usually, analyzing and controlling the emission of a random laser is difficult because light follows a random loop in a disordered medium. Here, an efficient method is proposed by inserting a silver nanoparticle at a specified position in the core area. It is found that emission wavelength/intensity of the laser changes correspondingly when the nanoparticle is set at different positions because the light trace transmitting in the cavity will be tuned by the nanoparticle (i.e., a typical characteristic that random lasing is sensitive to the surrounding small particles [18–20]). Based on this characteristic, position-dependent mapping of the random laser characteristics is revealed, which could be a significant means to microscopically controlled random lasing. In addition, as a unique feature compared with a conventional laser, the position-dependent output of the proposed random laser could be an efficient method for small particle identification and localization.
Sign up for Photonics Research TOC. Get the latest issue of Photonics Research delivered right to you!Sign up now
2. ANALYSIS RESULTS
Structure of the random microcavity laser is formed by three sections, as shown in Fig. 1. From outside to inside, the three sections are the outer layer that supports/protects the microcavity, the disordered layer that provides light scattering, and the core area that provides light amplification. Such structure could be realized using different materials, including polymers, semiconductor, glasses, etc. In our case, it is assumed to be realized by coating the inner surface of a glass capillary (its refractive index is 1.458, and its outer and inner diameters are 20 and 12 μm, respectively) with a disordered dielectric film (i.e., 2 μm thin film of mixture of nanoparticles and UV-cured adhesive with background refractive index of 1.5), and by filling the core with dye solution (i.e., Rhodmine B solution with refractive of 1.247), the so-called fiber-type random laser [19]. The numerical method to analyze the proposed microcavity is the finite difference time domain (FDTD) method, which is simulated by commercial software, i.e., FDTD solutions, taking perfect absorbing boundary conditions [21]. In the proposed cavity, light at the disordered layer will be scattered, part will escape from the cavity, and part will be fed back to the core. As a result, a large number of resonance modes can be formed through interference of multiply-scattered light. When the gain mechanism is applied, random lasing modes will emit, distributed nonuniformly in the core region. Here, we use the term “localization” to reflect nonuniform distribution of the lasing mode, which is different from Anderson localization.
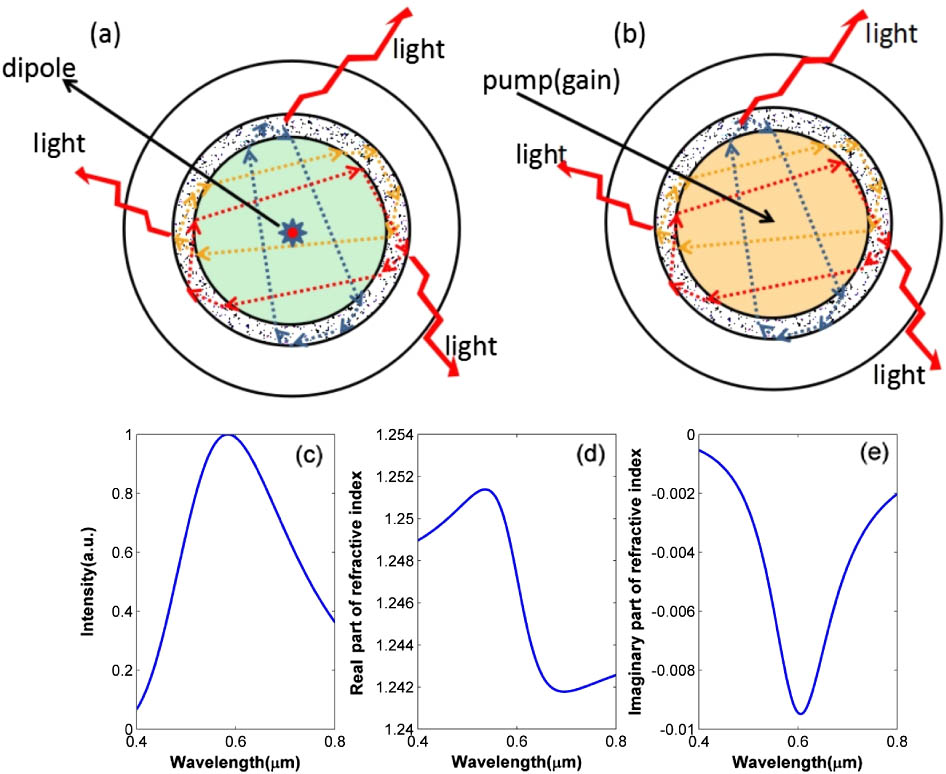
Figure 1.Sketch of the proposed structure. (a) Cold cavity. (b) Cavity with gain. (c) Emission spectrum of the dipole source. (d) Real part of refractive index of the gain region. (e) Imaginary part of refractive index of the gain region.
To analyze random modes of the microcavity, a wideband electric dipole light source [see Fig. 1(c)] positioned at the core is used to perform cold cavity analysis (i.e., gain of the core region is set to zero) in Figs. 2 and 3. In Fig. 2, the output is monitored at a specified point of the core region by a time monitor. Through fast Fourier transform (FFT), in analysis of the output waveform in time domain, randomly formed resonance modes in the core can be revealed [22,23]. Given the situation that other conditions remain unchanged, we change the scatter density to revise the scattering strength. Figures 2(a) and 2(b) show spectra of the cold cavity with scatter densities of 73.1 and , respectively. Comparison of Figs. 2(a) and 2(b) indicate that a few resonance modes become dominant when density of scatters increases because light will be confined more intensively within the gain region. As indicated by Fig. 2(c), the average intensity received by a monitor within the core becomes more dominate than a monitor outside the core.
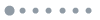
Figure 2.Output spectrum and integrated intensity of the laser from cold cavity analysis. In (a) and (b), the density of scatters is 2.3 and , respectively. (c) Average intensity received by an inner and outer monitor versus density of scatters.
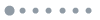
Figure 3.Intensity distribution for different resonance modes from cold cavity analysis; (a)–(d) correspond to mode resonance wavelength of 0.537, 0.562, 0.571, and 0.615 μm, respectively, as M1–M4 marked in Fig. 2(a).
Figure 3 gives the corresponding field distribution of specific modes supported by the cold cavity, at peak wavelength of 0.537, 0.562, 0.571, and 0.615 μm, respectively, which are indicated as M1–M4 in Fig. 2(a). It is observed that most of the light is confined in the core region with randomly distributed intensity due to interference of multiply scattered light. Besides, each mode has a unique intensity distribution with a few bright spots distributed randomly in the core region, reflecting spatial incoherence and localization of the confined light.
When gain is considered in the core region, random laser modes will selectivity emit. Figure 4 gives the spectra of four different monitoring points, C1–C4, with (the thick curves) and without (the thin curves) consideration of the gain mechanism. For clarity, intensity of the thin curves is enlarged 20 times. It is observed that, when gain is introduced, part of the resonance modes of the cold cavity will be selected by the gain profile [seeing Fig. 1(e)] and become dominate. For example, intensity of a few modes near the peak of the gain profile (i.e., around 600 nm) is enhanced several orders, which is a typical feature of lasing. Beside, the lasing spectrum varies remarkably for different monitor positions, indicating strong localization and spatial incoherence of the lasing system.
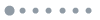
Figure 4.Lasing spectra from four different monitoring positions; (a)–(d) correspond to the monitor position of C1–C4, respectively. Taking center of the core as origin of coordinate, positions of C1–C4 are (0 μm, 6 μm), (6 μm, 0 μm), (0 μm, 6 μm), and (, 0 μm), respectively. The thick/thin curves correspond to the case of with/without the consideration of gain. For clarity, intensity of the thin curves is enlarged 20 times.
As we know, each mode of a random laser can be treated as a specific closed loop of light trace, which makes it emit at different collection positions. This characteristic provides a potential means to tune the laser output, while on the contrary, through the output characteristics we can localize the emission point. Hence, in the successive study, an efficient way to tune the random laser is proposed by setting an Ag nanoparticle at the core region, which could also be used for localization of the inserted small particle.
After inserting an Ag nanoparticle with a radius of 150 nm into the gain region, Fig. 5 gives the emission spectrum and field distribution at the maximum-peak wavelength. Here, the spectrum is monitored at point (0 μm, 6 μm). When the nanoparticle is set at position (0 μm, 5 μm), the emission spectrum has four peaks. For positions (5 μm, 0 μm) and (2.5 μm, 2.5 μm), both the spectra and the intensity distributions remarkably change. Because the dominant lasing mode is position-dependent, it can be tuned by setting the nanoparticle in a right position (i.e., where light scattering would change photon trance and interference related with the same mode). Figures 5(g) and 5(h) show maps of the peak wavelength and intensity distribution at the peak wavelength as a function of insetting location of the nanoparticle. It is observed that the peak wavelength randomly changes when position of the nanoparticle varies. These results suggest that the random lasing characteristics can be effectively tuned by changing localization of the nanoparticle. Meanwhile, position of the nanoparticle can be identified through combined analysis of the lasing wavelength and the intensity. In fact, when the nanoparticle is smaller than 110 nm, it will cause less intensity and wavelength change (e.g., the wavelength changes is less than 0.001 nm). Thus, the minimum size of identifiable particle is , suppose wavelength resolution of the system is 0.001 nm.
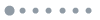
Figure 5.Output of the laser with an inserted nanoparticle. (a), (c), and (e) are the spectra; (b), (d), and (f) are the intensity distribution for the nanoparticle sited at positions (0 μm, 5 μm), (5 μm, 0 μm), and (2.5 μm, 2.5 μm); (g)/(h) is the mapping of peak intensity/wavelength of output when position of the inserted nanoparticle varies.
3. CONCLUSIONS
In summary, a simple structure of a microcavity random laser is proposed. Formation of resonance modes and output characteristics of the laser are revealed through cold cavity analysis and by introducing a complex refractive index for the gain material. Thanks to the decoupled gain and scattering regions, more light intensity and a few brighter modes will be trapped in the core with an increase of scatters in the cladding layer. Strong light confinement due to multiple light scattering gives birth to coherent random lasing with remarkable spatial incoherence, which makes the proposed laser sensitive to small particles even in the subwavelength scale. Taking use of this characteristic, efficient microcosmic control of the lase as well as a means of small particle localization were proposed. It is also worth mentioning that the proposed platform is a fiber-type cylindrical waveguide incorporating a microflow of dye solution, which could also be introduced to semiconductor films, traditional optical fibers, or biology samples/cells for applications of light generation and optical sensing.
References
[1] D. S. Wiersma. The physics and applications of random lasers. Nat. Phys., 4, 359-367(2008).
[2] J. Andreasen, A. A. Asatryan, L. C. Botten, M. A. Byrne, H. Cao, L. Ge, L. Labonté, P. Sebbah, A. D. Stone, H. E. Türeci, C. Vanneste. Modes of random lasers. Adv. Opt. Photon., 3, 88-127(2010).
[3] J. Liu, P. D. García, S. Ek, N. Gregersen, T. Suhr, M. Schubert, J. Mørk, S. Stobbe, P. Lodahl. Random nanolasing in the Anderson localized regime. Nat. Nanotechnol., 9, 285-289(2014).
[4] H. Cao, Y. G. Zhao, S. T. Ho, E. W. Seelig, Q. H. Wang, R. P. H. Chang. Random laser action in semiconductor powder. Phys. Rev. Lett., 82, 2278-2281(1999).
[5] E. S. P. Leong, S. F. Yu, S. P. Lau. Directional edge-emitting UV random laser diodes. Appl. Phys. Lett., 89, 221109(2006).
[6] C. J. S. de Matos, L. S. de Menezes, A. M. Brito-Silva, M. A. M. Gámez, A. S. L. Gomes, C. B. de Araújo. Random fiber laser. Phys. Rev. Lett., 99, 153903(2007).
[7] R. Polson, Z. Vardeny. Cancerous tissue mapping from random lasing emission spectra. J. Opt., 12, 024010(2010).
[8] H. K. Liang, B. Meng, G. Liang, J. Tao, Y. Chong, Q. J. Wang, Y. Zhang. Electrically pumped mid-infrared random laser. Adv. Mater., 25, 6859-6863(2013).
[9] D. V. Churkin, S. Sugavanam, I. D. Vatnik, Z. Wang, E. Podivilovb, S. Babin, Y. J. Rao, S. Turitsyn. Recent advances in fundamentals and applications of random fiber lasers. Adv. Opt. Photon., 7, 516-569(2015).
[10] Z. Hu, Q. Zhang, B. Miao, Q. Fu, G. Zou, Y. Chen, Y. Luo, D. Zhang, P. Wang, H. Ming, Q. Zhang. Coherent random fiber laser based on nanoparticles scattering in the extremely weakly scattering regime. Phys. Rev. Lett., 109, 253901(2012).
[11] F. Antenucci, A. Crisanti, L. Leuzzi. The glassy random laser: replica symmetry breaking in the intensity fluctuations of emission spectra(2014).
[12] W. L. Zhang, Y. J. Rao, Z. X. Yang, Z. N. Wang, X. H. Jia. Low threshold 2nd-order random lasing of a fiber laser with a half-opened cavity. Opt. Express, 20, 14400-14405(2012).
[13] E. A. Zlobina, S. I. Kablukov, S. A. Babin. Linearly polarized random fiber laser with ultimate efficiency. Opt. Lett., 40, 4074-4077(2015).
[14] X. Du, H. Zhang, X. Wang, P. Zhou, Z. Liu. Short cavity-length random fiber laser with record power and ultrahigh efficiency. Opt. Lett., 41, 571-574(2016).
[15] J. W. Merrill, H. Cao, E. R. Dufresne. Fluctuations and correlations of emission from random lasers. Phys. Rev. A, 93, 021801(2016).
[16] R. Sarma, A. G. Yamilov, S. Petrenko, Y. Bromberg, H. Cao. Control of energy density inside a disordered medium by coupling to open or closed channels. Phys. Rev. Lett., 117, 086803(2016).
[17] B. Bhaktha, N. Bachelard, X. Noblin, P. Sebbah. Optofluidic random laser. Appl. Phys. Lett., 101, 151101(2012).
[18] A. Consoli, C. López. Decoupling gain and feedback in coherent random lasers: experiments and simulations. Sci. Rep., 5, 16848(2015).
[19] W. L. Zhang, M. Y. Zheng, R. Ma, C. Y. Gong, Z. J. Yang, G. D. Peng, Y. J. Rao. Fiber-type random laser based on a cylindrical waveguide with a disordered cladding layer. Sci. Rep., 6, 26473(2016).
[20] Q. Song, S. Xiao, Z. Xu, V. M. Shalaev, Y. L. Kim. Random laser spectroscopy for nanoscale perturbation sensing. Opt. Lett., 35, 2624-2626(2010).
[21] X. Li, S. Yu, A. Kumar. A surface-emitting distributed-feedback plasmonic laser. Appl. Phys. Lett., 95, 141114(2009).
[22] H. Cao. Review on latest developments in random lasers with coherent feedback. J. Phys. A, 38, 10497-10535(2005).
[23] C. F. Bohren, D. R. Huffman. Absorption and Scattering of Light by Small Particles(1983).